Efraim Racker

The Great ideas of Biology
Transcript
[Inaudible Discussion ]
- >> Richard Cerione
Good evening.
I like to welcome all of you to the Efraim Racker Lectureship in Biology and Medicine and as has been the case every year.
I'm especially pleased to welcome his daughter Dr. Ann Costello and her husband John.
This lectureship was initiated in 1992 with the intention of bringing close to the scientific community and into the general public examples of the major developments in biology and medicine by those individuals who have had extraordinary impacts in these areas and in this regard, we've been quite fortunate in having had some truly outstanding lecturers in the past, many of them have been Nobel laureates including our first lecturer Jim Watson, our second lecturer Sydney Brenner as well as David Baltimore, Harold Varmus and of course tonight's lecturer Paul Nurse.
What we strive for here is to try to identify individuals whose contributions to scientific impact truly epitomize the breadth of Ef's research interest in the fundamental contributions that Ef's laboratory made throughout the years to basic biochemistry into a molecular understating of human disease.
After a distinguished career in New York University and then at the Public Health Institute of New York, Ef came to Ithaca in 1996 as the Albert Einstein Professor of Biochemistry and as the Chair of the then-section of biochemistry here at Cornell.
He was truly an inspiration to a number of faculty colleagues as well as many students and postdoctoral associates who had the privilege to train with him, many of whom would then go off and start their own accomplished independent careers.
And I can tell you from first hand experience he was a wonderful friend and a terrific mentor.
Ef did science as if were an art form with the marvelous style that relied-- everything is lodged on vision and on intuition as it did on technical knowledge and literature details which probably comes down as a surprise to many who knew him because if you knew him, you knew that one of his other great loves was art and painting and indeed throughout his life, Ef would combine art and science in a seamless fashion and he was absolutely [inaudible] for both of those arenas.
Ef's scientific interests were directed in understanding the fundamental steps of a variety of biochemical pathways including oxidative phosphorylation, neurotransmitter release, the regulation of enzyme catalysis, and the molecular mechanisms underlying the control of cell growth and how the loss of that control can lead to cancer.
And he had this marvelous unique ability really to go back and forth between the most basic fundamental concepts in biology and then link those to what were the most impressing and interesting biomedical questions.
Basic research had no greater champion than Ef and as many of you who knew him can attest, he had a real presence that we've yet really be able to replace here at Cornell.
However, our hope is that the spirit of Ef's scientific approach is love for basic research and how fundamental discoveries can be applied to alleviate disease and human suffering will continue on through this lecture series.
And so I'm particularly delighted to be welcoming Dr. Paul Nurse tonight who will be introduced by Dr. David Shalloway.
[ Noise ]
- >> David Shalloway
Reproduction is one of the most fundamental characteristics of life.
Now the cellular level reproduction means cell division.
Thus it is that the cell division cycle, cell cycle for short, plays such a central role in basic biology.
Cell cycle research is one of the classic and oldest areas of cell research dating back essentially to the advent of microscopy and the word 'mitosis' referring to the separation of duplicating chromosomes was coined in the 1880's.
But it wasn't until the 1980's, a hundred years later, that the molecular mechanisms regulating this process began to be uncovered and some of the key discoveries in this arena were made in the laboratory of our Racker lecturer tonight, Sir Paul Nurse.
Disruption of the cell cycle regulation leading to unbridled cell replication is the key feature of cancer.
So this area was of intense biomedical interest as well.
I still remember the great excitement when the Nurse group showed that one of the key regulators of the yeast cell cycle was the protein kinases.
Since kinases, which regulate other proteins by adding phosphate groups to them, had already been shown to be important in the initiation of cancer and frankly, we were amazed when it showed that a human cell division control gene could functionally replace the yeast gene in one master stroke "identifying and cloning" the human gene while simultaneously showing that the molecular mechanism of control was extraordinarily conserved across species.
It was a great moment in biology.
And I want to take up time telling you details that you can read in the program.
Suffice it to say that Paul Nurse has been honored in many ways for his scientific accomplishments, among others, the Lasker Award in 1998, knighthood in Brain in 1999, and the Nobel Prize in 2001 and extending his contributions outside the laboratory while maintaining his research activities, he has gone on to play a major leadership role in science as Director of the Imperial Cancer Research Fund, the Chief Executive of Cancer Research United Kingdom, and since last year as the President of Rockefeller University in New York.
We are delighted to hear him speak tonight on the great ideas in biology.
[ Applause ]
- >> Paul Nurse
Well, thank you very much David to that very nice introduction.
I have to say it's a real pleasure to be here.
I've never been to Cornell before.
I'm looking forward to doing some walking here over the weekend as long as it doesn't rain, of course.
[laughter] It's also an honor to be giving this lecture.
I had the privilege of meeting Professor Racker when I was a post doc in the '70s.
I was trying to remember quite where.
I think it was in a Gordon conference and I remember him very clearly.
And so, it really is a pleasure to be giving the Ef Racker lecture and to meet his family earlier this evening.
So thank you for this honor to be here and to have the privilege to talk to you all.
I wasn't quite sure what to talk about but I decided in the end not to talk about anything that I have done myself or my laboratory's done but to talk about something which I perhaps rather pompously call the great ideas of biology and I'm going to cover a number of things this evening.
There will be some signs, there'll be some history, there'll be some speculation certainly towards the end, and there will be just a touch of philosophy.
I'm rather hoping that this will cover everybody's interest in the audience this evening.
Now biology is really a subject that deals in details.
You know, biology's like meticulous, the sorts of things we do are, you know, we list species in a habitat or we count the numbers of hairs on a beetle's legs or maybe if we're molecular biologists, we sequence genes.
You know, it's much the same thing as counting hairs on beetles' legs.
[laughter] And we, on the whole, don't have so many great ideas and grand theories, certainly unlike the physicists for example but I thought I would look to see what, in my view, were the great ideas of biology and talk about those and I'm going to talk about four ideas, I've listed them up here, which I want to explain the development of and explain their significance this evening, which I think are indeed great ideas of biology and then I thought I'd end with a fifth which is yet quite to make it and maybe won't make it but I'd like to suggest that it is indeed important.
And I think when you put these ideas together, you have a better sense about the nature of life and that's really what I want to try and communicate to you this evening.
Well, what are these ideas?
Well, the first idea is the idea of the cell, the cell doctrine and in fact this is really quite an old idea.
It can be simply stated that all life is composed of cells and the cell is the simplest unit exhibiting the characteristics of life.
So it's as if you're like life's atom, the basic unit of life.
Now it's an idea that took about 200 years to become fully developed and it began in the 1660's with Robert Hook who was experimentalist to the royal society.
That's sort of the old scientific society, the National Academy of Britain, invented in the-- or setup in the 1660's, and Robert Hooke was employed as an experimentalist.
He was actually basically for the aristocrats who didn't dirty their hands doing experiments and Robert Hooke was hired to dirty his hands doing the experiments and he used the microscope and this is, in fact, a picture of his microscope.
He worked in Oxford which is where my home is, my family home, and he used his microscope to look at living things.
That's a very famous picture of a louse that some of you may be familiar with.
And he, during these investigations in the 1660's, took a thin slice of cork and this is what he saw.
And in that cork, he saw lots of little sort of boxes, they reminded him of a monk's cells and that's why he called them cells and this was the first description of a cell.
It also exist-- of course demonstrates that old principle that, as it's so often the case in science, technology begets discovery and it was the invention of decent lenses in the 17th century from opticians and then on the development of first the telescope which gave Galileo the Jupiter's moons and really auctioned in the Copernican revolution and with Hooke and others as I shall describe, the microscope which was so important to biology.
Once the cells have been seen, then everybody jumped on the bandwagon, just like science today, of course.
[laughter] And people turned their attention to biological material and started producing very beautiful pictures and engravings and you'll see here a picture that came from somebody else also from England, [inaudible] and this is, in fact, a section of a vine stem and for those botanists in the audience, you'll see that it's actually a very good representation of plant anatomy.
The microscope was also used not only to discover cells as the basic unit of living things that you could see but in the hands of Leeuwenhoek, a Dutch draper, to discover that there were microorganisms that nobody had ever seen before and he was the first to described bacteria and this is a picture of what he saw actually when he took a scraping of his teeth and incubated them in liquid and these are the first descriptions of bacteria.
He called them animalcules that it's like small animals.
Now Leeuwenhoek, I'm rather attracted to his character because he was a great experimentalist.
He was, as I've said, rather a draper of humble origins.
He lived in Delft, in late 17th century Holland and he did his work, he did his drawings, he sent them to the Royal Society and produced many thousands of illustrations, was eventually elected as a fellow at the Royal Society.
We don't really know what he looks like but I thought I'd put it in the following picture because Ef Racker was so interested in art and this is a picture actually from Vermeer, it's in the Louvre and Vermeer did two paintings of scientists, a geographer and astronomer and this is one of them and it's the same person and interestingly, Vermeer lived in Delft and Leeuwenhoek was an executor on his will.
So they certainly knew each other.
And I like to sort of just sort of fantasize that maybe this is a picture of Leeuwenhoek, the scientist, who he drew here and it's probably not true but let's just imagine [laughter] that it might be true.
So by about 1700's, cells have been described, single-celled organisms have been discovered, blood cells also discovered by Leeuwenhoek, Swammerdam, Malpighi had also discovered blood cells and so, there was a sense that cells were important.
Animal tissues were rather refractory to analysis.
They're not so easy to fix into section and to look at under the microscope and in fact, it took improvements in those techniques and improvements in optics until by around 1800 or thereabouts, it was clear that cells were found universally in animals as well and so seemed to be emerging as a fundamental unit of life.
And it was around 1800 that that became furtive art and I think there are reasons for that and I think that there are reasons for that.
It was also at the same time that chemists were thinking about-- and physicists were thinking about the basic unit of matter and experimental work which is, of course, a very ancient idea from the 4th century B.C. but at this time in 1800, doctors and others were providing experimental evidence in favor of atoms and they were thinking about the basic unit of matter and it is no surprise that biologists began to think about the basic unit of life.
Until around the 1830's, Theodor Schwann and his compatriot Schleiden, the botanist, Schwann was a zoologist, postulated the cell doctrine.
This is the picture of Schwann, a rather serious looking German and there will be a number of photographs of serious looking Germans actually in my [laughter] talk tonight.
Usually their beard, in this case he's not.
And they proposed, Schleiden and Schwann, in this nice quotation here translated into English essentially the basic premise of the cell theory.
We have seen that all organisms are composed of essentially like parts, namely of cells and that these cells are formed and grown in accordance with essentially the same laws, hence, that these processes must everywhere result from the operation of the same forces.
Actually, I should refer to David's introduction because this was really a statement about the universality of the control of cell division which took us another 150 years to actually establish.
But he clearly recognized the universality of the-- of this sort of process right at the very beginning of the cell theory.
Schwann and Schleiden recognized the cell as the basic structural unit of life and but it took a few more years before it was suggested that it might be the basic functional unit of life as well.
And this was proposed by the founder of pathology, Rudolf Virchow, in the mid 19th century and he proposed two statements that are very important in the 1850's.
Every cell appears as a sum of vital units, each of which bears in itself the complete characteristics of life.
This is the statement that the cell is the basic unit of life.
It's the simplest unit that has the characteristics of life.
It's a very, very important idea.
It's one that I don't think has sufficient publicity compared with a couple of the later ideas that I will talk about.
He also, and in this second quotation here, clearly identified that cells arise from pre-existing cells.
This was still the time when vitalism was rife and this clearly put the case that cells only emerged from pre-existing living cells.
Now in my view, this is a real milestone in biology, not as I've said really properly recognized.
Now why is it such a milestone?
Well, first of all, cells are the basic unit of life.
Second, their growth and development really form the basis of the growth and development of all living things.
Here's a picture of an embryo, a mammalian embryo.
If you look carefully, what you can see are little dots that make up that embryo.
They are the cells.
This embryo has been formed by process of failure with this one.
No what you would've seen here is a round of cell division.
So now you have to be very imaginative.
[laughter] On the left hand side, as you can see on this slide, there is a single cell.
[laughter] As you proceed to the right, you will see that single cell divide into two, into four, into eight and into 16.
I think it's very evident by this abstract illustration.
[laughter] And this shows that the process of formation of an embryo is a consequence of repeated cell divisions and that will generate the embryo that I showed you a little earlier.
When I talk to undergraduates in their first year who are getting a little bored with my lectures, I usually put this slide up.
This is a mammalian egg being bombarded with sperm.
Well, apart from getting mildly excited by sex and all of that, [laughter] I can also point out to the students this: that everyone of you once was a single cell like this.
So you should be interested in cells [laughter] 'cause this is what you all were at one time in your life as was every other living thing on this planet.
So that's my first great idea, the cell, the cell doctrine, the basic structure and functional unit of life.
Now my second great idea, the gene, and this is one which I think you will be more familiar with.
It's one that has greater publicity and I want to explain a little bit about the gene, particularly where the idea came from.
So let's first of all just summarize what I mean by the idea of the gene.
What's crucial here is the sense that genes, which are the basis of heredity, that is why offspring resemble in some way their parents or their grandparents, that genes which are responsible for that-- those sharing characteristics can be considered essentially as particles, particles of information that are passed on from one generation to the next and that these particles encode information that is important for the development and subsequent reality of a living organism, and they determine how that organism actually turns out.
And the important issue here is that they are particles which are unchanging and this is an idea that was generated in the 1860's, and I'll explain why, and that was in a time when most theories of inheritance were not based on particles of unchanging information but had some sort of blending quality.
Darwin, who I shall talk about a little later, thought for example that there was a blending of hereditary information from mom and dad to generating the offspring.
Where did this idea come from?
It came from a rather obscured town now in the Czech Republic called Brno and it involved a monk, Gregor Mendel and he was an extraordinary man.
Mendel was trained, in fact, as a physicist.
Initially as a meteorologist, he was the first to describe the massive drop in air pressure during a thunderstorm, during a major cyclonic thunderstorm and his training in physics is thought to given him a quantitative bent 'cause on the whole, biologists don't like counting things, you know?
[laughter] We generally like to sort of describe things.
We sort of-- you know, with the soft end of the sciences.
[laughter] You know, when I was a school boy in the 18-- 1860, in the [laughter] 1960's, this talk is beginning to get to me actually.
In the 1960's, you know, it was the bright boys who did physics and chemistry and the slow ones-- I went to a boys' school, of course.
The slow ones did biology 'cause they could draw things under the microscope, okay?
[laughter] But what Mendel brought to this was the idea that you should count things, you should quantitate them.
And he worked in a monastery but he didn't generally monkish things, okay?
[laughter] I've been to this monastery.
It's actually got-- it's a very big monastery with a very large garden and he had a huge greenhouse built for experiments.
His abbey was perfectly happy to him to do experiments.
It was a sort of intellectual order.
And he had a big greenhouse built about 30 feet by 20 feet and he bred peas amongst other plants and he did a number of experiments and chose peas in the end because I think they produced results that he could quantitate and what he tended to do was to cross pea plants with different attributes, different qualities, some which were very tall versus dwarf versions, different colored flowers, different shaped seeds and he had clear characteristics which he then counted in how many seeds were produced and what characteristics the plants had which were grown from those seeds and he noticed a couple of things.
The first he noticed, and this was not just Mendel, it had been discovered previously but nobody made any sense of it, is that if you were to cross two plants with different attributes, then often the attribute of one of those plants would disappear in the first generation and then, however, if you then cross those plants with each other, the original phenotypes as we call 'em, characteristics of the grandparents would reemerge in the subsequent offspring.
This was known in sort of popular sort of family histories, that a child would resemble in some ways their grandparents.
So this was sort of in the law but he really noticed it and thought about it.
The second thing he did was to count, as I've already stressed, the numbers of plants that were produced and when he did that, he realized that certain ratios emerged and that had never been noted before.
People have noticed the qualities but not the quantities that emerged.
And simple ratios began to emerge: 3 to 1, 9 to 3 to 3 to 1, 1 to 2 to 1, and he realized that partly because of his mathematical background as a physicist that these resembled simple equations that he could think about in his head and he proposed that the reason for these simple ratios was because each plant had two particles that would be important for determining a particular feature or attribute.
Every time they produced a gamete, the equivalent of a sperm and an egg, then each sperm or egg, if this was an animal, would receive one of those particles.
Then, on fertilization, two particles would come together again to produce a new offspring and the final attributes that were associated with the offspring were determined by the interaction between those two particles.
So for example, if you were to have a tall and a short plant, let's say, when you cross them together, you would end up with a set of offspring, all of which were tall but when you crossed all those tall plants with each other, you'd get a simple ratio of plants that came out 1 to 3 that were short and tall and that could be explained by a particle theory of gene basis, with genes determining the information that would determine what those plants were like which were passed down in this sort of way.
This, frankly, was a brilliant piece of reasoning.
Indeed, it was so brilliant that nobody took the slightest piece of notice on it.
[laughter] He published it in 1865.
It was in a Czechoslovakian journal but it was one that was widely read in Europe.
He sent, for example, a copy to Darwin.
Darwin had this paper in his library.
It was uncut, he'd never opened it.
[laughter] Mendel's work was referred to in the Encyclopedia Britannica in the 1880's but nobody took any notice of what he said.
Then suddenly in just at the turn of the 20th century, three different groups of scientist rediscovered Mendel and his theories were completely accepted.
Why was that?
Why did it take 30 years to move from nobody taking any notice to suddenly being accepted?
Well, one cannot be sure of that, of the reason for that but I'd like to speculate why and I think one reason is this: that Mendel's reasoning was abstract.
He counted things and he determined something from that, it was an abstraction.
He didn't have an easy, concrete realization of what it was that he was, in fact, talking about.
In the subsequent 30 years, from 1865 up to 1903, microscopic work dramatically improved and people, Fleming and Strasbourg amongst them, described as we've in fact heard from David, mitosis and the existence of chromosomes and under the microscope, they saw that every cell division, there appeared string-like structures, chromosomes, which separated into the two newly dividing cells and they noticed that these chromosomes, see these strings seemed to split down the middle.
More importantly-- and I'll just show you, this is a typical example, this is a late 19th century plant tissue.
It's an unnamed root actually, and you can see the string-like characters I'm talking about.
More importantly, when people turned their microscopes on to gamete formation, producing eggs and sperm, they noticed that the numbers of chromosomes that were generated during gamete formation were reduced from-- to half the number.
So if there were 10 in the original organism, there were only five in the gamete and this was found initially in a nematode by van Beneden, this is his early illustration, where there were only four chromosomes.
So it was very obvious 'cause you could see the number difference very clearly.
So suddenly, there was a reduction in the numbers of chromosomes in the process we now know to be miosis which form gametes.
Suddenly, there was a concrete realization of Mendel's abstract reasoning 'cause now you could argue that the chromosomes behaved like Mendel's genes.
There were two and they will reduce in number every time a gamete was formed and then they reform to make a zygote which consisted of two again, exactly as Mendel postulated with genes and it was only a few years before it was proposed that chromosomes were strings of-- like beads on a string of genes and I think it actually reflected the fact that the abstraction, this brilliant piece of reasoning for Mendel could be brought on a concrete reality.
So this resulted in the idea of a gene and of course now we know from studies, first of all, I have say from the Rockefeller where DNA was discovered as the genetic material by a trio of workers, one of whom was Mac McCarty who published his paper in 1944.
This was with the pneumococcus transformation experiment for those familiar with this in the audience.
And one of the most extraordinary events of my last year is to meet Mac McCarty who's still an emeritus professor of 93 in the Rockefeller when I'd read his papers in the late 1960's and I thought he was dead then.
[laughter] And the guy is truly extraordinary.
He stills comes to seminars, you know?
And he's 93.
He's a living a living legend.
Anyway, he is less well-known and of course Watson and Crick, and Watson gave the first of these lectures, who determined the structure of DNA, the consequences of which are important both for understanding how information is encoded within a gene, and I should say something a little bit more about that later, and how it's precisely replicated when the double helix is unwound and the string of letters that make up one strand of DNA is copied in a complimentary fashion to produce a new double helix and that's represented here, this symbolic picture here of replicating DNA.
And the important point about this is that this happens every time a cell reproduces.
It's the first key event that DNA is replicated.
The second key event being that these chains here are separated into the two newly divided cells by the process of mitosis and here we just see an example of a time lapse series of photographs of a cell undergoing mitosis and the separating chromosomes nicely seen here in the bottom left, separate into the two newly divided cells.
So this tells us the nature of the gene and how it is reproduced such that each newly divided cell will receive a full compliment of genes and therefore, their hereditary material.
And this was the beginning-- the structure of DNA and the discovery of DNA was the beginning of the molecular biological revolution of the second part of the 20th century and central to that was the central dogma which is illustrated here in which was so well-advertised by Francis Crick, of course Jim Watson's colleague, where we see that DNA is transcribed into RNA, also a nucleic acid, also made up of a group of letters, and then translated from nucleic acid into protein where protein is a string of amino acids, the basic building blocks of proteins and sequence of those amino acids determines the shape and the qualities of the protein which then determines the characteristics of how the cell works because the way a protein folds up and the way in which charge is distributed throughout that protein determines the characteristics of how a protein works in catalyzing reactions as enzymes, and also structures and this forms the basis, the central dogma here, of how genes encode information.
So that's my second great idea, the gene as the unit of heredity.
So what's my third?
Well, my third is probably the one that is best known to the public and possibly the most notorious, at least in certain sectors of the public [laughter] and that is evolution by natural selection and to be honest, in my view, this is probably the most beautiful idea of biology and if I have to choose any, this would be my-- the one that I think is the greatest idea of biology.
Very simply stated but so fundamental to understanding life.
Now this idea was developed, of course, by Charles Darwin and this is a picture of Darwin in the 1880's, another bearded Victorian gentleman and who was very reluctant to publish this idea.
He first had it in the 1840's, wrote it in his notebooks but he needed a competitor before he would eventually publish it which he did in 1858.
That competitor was Arthur Wallace who had the same idea, was recovering from a fever working in Borneo.
He was a collector of animals and plants in Borneo.
He had the same idea and sent it in a letter to Darwin and Darwin noticed it was the same idea as he's had for 15 years which he'd been reluctant to publish because of its implications for-- particularly for the church and his wife being devoutly religious.
He was very bothered by his sets of theory.
But both being tremendous gentlemen, they agreed to publish together and did in 1858 and then in 1859, Darwin published the 'Origin of the Species' which is a 500-page book describing all his thoughts of the previous 15 years.
Now Charles Darwin, of course, was a great biologist but I want, in thinking about him, to make some reference to his grandfather.
His grandfather was Erasmus Darwin who lived in the period about 1780, active from 1780 to about 1810 and I want to mention him because he also proposed evolution because evolution was not Darwin's idea.
Darwin's idea was the-- was carried out by natural selection.
The idea itself has its origins probably with Aristotle, in fact, who had a crude idea of evolution.
But Erasmus really pushed it and he published all his scientific results.
He was a member of the Lunar Society which some of you may have heard of.
The Lunar Society was a collection of eccentric English scientists who would meet once a month at the time of the full moon, [laughter] was thought to be possibly the origin of the lunatics, okay?
[laughter] The term lunatics.
Largely, so they could write home at night and see where they were going and they would come and discuss scientific discoveries and technology discoveries.
Watt, you know, James Watt of the steam engine, for example, was a member of the lunatics and others, Wedgwood who was another one, of pottery fame.
And Erasmus Darwin was a member of this, a leading member and he was a physician who lived in Lichfield, the Cathedral town just known for Birmingham, and he was a very charming man with a great stutter, a very large, fat man who was-- had a charming conversation.
He was well-known for being a physician who would only charge people who could afford to pay him.
So he's very popular because he treated for free those who couldn't be-- he couldn't be-- who couldn't afford and-- to pay for his services and because medicine was moderately primitive at that time, he was most famous for being able to predict whether a patient would die or not.
[laughter] So this apparently was very important because if you were told with some reliability that you were going to die, then you'd put your house in order and that was very important at that time and if you were told you were going to recover, then you didn't have to go through the embarrassment of deciding exactly who would inherit what.
And so he was, in fact, well-respected.
The difficulty was he was such an enthusiast for evolution that he'd put on his motto on the side of his coach which was in Latin which I can't remember, not being very good at Latin, but translated was, "Everything comes from shells." They argued that shells was the most super simple and primitive form of life that everybody knew about, and everything came from that.
But the Dean of Lichfield Cathedral didn't like it and because Erasmus lived in Cathedral Close, his house actually just overlooks the Cathedral, the Dean began to preach against him and Darwin lost all his wealthy patients.
So he had to compromise and he took his motto off and got all his patients back.
So that's a lesson for us all.
[laughter] We got to respect or paymasters, of course.
But Erasmus had a grandson, Charles, who put his own-- oh, one other thing about Erasmus.
Two other things, actually I like Erasmus as you can see.
He wrote all his scientific theories in poetry.
[laughter] So all his books are in poetry.
I mean really, he's truly a lunatic actually.
I have-- I actually have some 18th century copies of his books.
They are all poetry, blank verse.
He was, in fact, better known as a poet than as a scientist at the time and for a while was known better than Shelley and Keats and co. He was immediately eclipsed by the romantics, however, 20 years later.
The second thing he did is that he had a number of illegitimate children by his housekeeper in between he stood one.
It's-- and he didn't quite know what to do with them.
He had two daughters and he decided they should become school teachers for girls and he established the first girls' school in England and he wrote a book on female education which he gave to his two daughters.
They were the first teachers in a female school in England.
So he's an extraordinary character, a very sympathetic character.
Back to Charles.
[laughter] Now Charles' idea was developed, and I'm going to have to summarize what are the features.
Well, how-- what are the features of living things that bring about evolution by natural selection and there's qualities that have been identified and they were first really cleared stated by Muller, a very famous geneticist, and he stated this I think in the-- he thought about this in the '30s but I think he stated it most clearly in the '60s.
And he identified three features which he felt defined evolution by natural selection, the characteristics that a living organism must have to evolve by natural selection and indeed, he went further to say anything that has these three features is defined as living.
You don't ask the question, "Do you have DNA protein on you?" If that's that, you ask do they have these characteristics?
And these characteristics were: all living things must reproduce.
Secondly, all living things must have a hereditary system whereby information, defining how that living organism operates, is copied and inherited during that process of reproduction, okay?
So that's a system of hereditary.
And the third feature, if that hereditary system must exhibit variability and that variability must be inherited.
If an object has those features, it will evolve by natural selection and that is-- it's truly a historical definition of life but it will define what life is.
Now interestingly, those exact characteristics are what happens every time a cell reproduces.
A cell, as I've told you, has chromosomes made of genes which form the basis of a hereditary system.
Cells reproduce by cell division and that hereditary system works by passing on genes to the two newly divided cells.
The genes are made up of a sequence of nucleotide letters.
There are errors in the copying of those genes which introduces variability.
So when the cell divides, there's potentially variability.
This means that every time a cell divides, it has the characteristics necessary to evolve by natural selection.
This would have been seen in primitive life when it was unicellular.
It is also seen in the cells in our own body which undergo the changes that generate cancer, which is another example of evolution essentially by natural selection where you generate genetic changes that lead to cells dividing more rapidly, growing more rapidly and as a consequence, generating cancer.
It's a very important concept underlying very much about how life works.
Now that's the third idea and it's the one that you will have heard a lot about because there's a whole industry of popular scientific publishing around Darwinian-- around Darwinian theory and it's also, of course, very contentious with certain people.
But there is something inadequate, unsatisfying about defining life in this sort of way.
Defining life is a sort of historical process of characteristics that give rise to natural selection, somehow loses the extraordinary behavior of what life is.
This is extraordinary in its own right but you lose the fantastic characteristics of life of a living organism and everything that's associating with a living organism.
To understand that, I want to move to my fourth idea and that is the idea that life should be understood in terms of chemistry and that's the fourth established idea I want to talk about today.
Now this has its origins from the late 18th century from studies of fermentation and I built my career on yeast which undergoes fermentation and I'm very pleased to see that this idea has its origin also in studies of yeast.
And the first person who contributes to this story is not the man here who is Louis Pasteur but another Frenchman, Lavoisier the Chemist.
Now Lavoisier was a fantastic chemist who contributed many basic principles to chemistry.
He, unfortunately, was also a tax collector and as a consequence of that, he lost his head during the French Revolution because they didn't like tax collectors by 1793 and he was guillotined.
The Rockefeller used to have a fantastic picture from that time of Lavoisier from Davy but in former harder times we had to sell it and it's now in the Metropolitan Museum of Art in New York.
[laughter] I should actually get a picture of that here too.
Now what did Lavoisier notice?
He, being French, was interested in wine and asked what happens in fermentation and he proposed that what happens in fermentation was chemical transformation, the changes of certain chemicals into other chemicals and that's what was happening when we were seeing fermentation.
Now this idea or thought was developed by Louis Pasteur, this painting that we have up here, who also studied wine.
And this is another example of the paymaster calling the tune because the fact was the wine industry in France underwent periodic crises when wine became spoiled and they wanted to know why and they paid Pasteur money to try and find out why.
So he examined rotten, spoiling wine and compared it to normal wine and looking at it under the microscope realized that, in fact, there were different microorganisms in spoiled wine compared with normal wine and that he then postulated that the chemicals which damaged the wine and gave it a bad taste came from these strange organisms that were bacteria that was found in the spoiled fermentation.
And he thought a bit more about that and then began to conclude that specific fermentations, that is, the generation of specific chemicals was due to specific microbes.
So, different microbes were producing different chemicals.
And then he postulated that maybe it was a physiological act that yielded these different chemical products and this physiological act was important for the life of the cell.
In other words, chemistry was important for how cells worked.
That was a really important insight because it began to push away the idea of vitalism, that is, the idea that something was special in living things that did not obey the laws of physics and the laws of chemistry.
Here, the miraculous changes that we saw during fermentation, simple as they are compared with how living things worked, could be understood in terms of chemical changes.
The next insight that occurred in the second part of the 19th century was to demonstrate that these different chemicals were formed by proteins called enzymes, of course, which act as like little chemical factories which because of their shape and the charge on those shapes that bend the chemicals bonds and change one chemical into another.
And they postu-- and this work was postulated that there were enzymes that were found in living things that carried out these chemical transformations and importantly, that you could extract these enzymes and they could work in a test tube, in vitro, in glass and this was worked out by the Buchner Brothers here in about the 1890's.
And they then proposed that an extract, and they did it also from yeast, extracts of yeast contained enzyme catalysts which carried out the chemical reactions that made up fermentation and this led to a cornerstone of biochemistry and that is that life's processes are reducible to chemistry and that chemistry is responsible for metabolism and growth and to put that together with the theory of the gene, of course, those enzymes are coded by genes.
And we see this now producing this sort of picture of how life works.
Over the subsequent years, this was fully recognized until Loeb, another Rockefeller scientist, proposed that the cell should be considered-- in 1910, the cell should be considered as a chemical machine.
Now it may be a chemical machine but it is a completely extraordinary chemical machine.
Why is that?
Well, I now want you to think about cells in maybe a different way than you've done before.
What you have to imagine is that if chemistry is the basis of life and how a living cell works, then chemical-- many, many hundreds, indeed thousands of chemical reactions are going on in a cell at the same time.
Now, that is only possible if you have many hundreds, indeed thousands of individual chemical microenvironments which allow those chemical transformations to take place, individual enzymes, which sit in a particular microenvironment of pH, with certain ions, with certain substrates which allow those chemical transformation to take place.
How does that come about?
It comes about by an exquisite compartmentation that makes up the cell and I've illustrated that here and this shows that I've never yet mastered computer drawing, I have to say.
[laughter] So what I do is sort of use little colored pencils and then scan it into the computer, okay?
My students just mock me, I have to say.
And what I just want to illustrate here is what looks like sort of a child's picture is and indeed should be considered as such, [laughter] is a cell illustrating the chemical microenvironments which make up that cell.
First of all, you have to isolate with a membrane the cell from the rest of the world, okay?
So you can do special things.
Then imagine different little environments.
Here's a sort of-- here's an enzyme and that's a little environment.
It's a single protein and it has a certain shape and a cleft which isolates you from the environment.
You can put a couple of proteins together and make that environment slightly different.
You can bound it with a membrane in an organelle to separate that environment from the rest of the environment of the cell.
You can put together different enzymes that channel substrates and products down through a series of enzymes to go from A to B to C to D to E and therefore generate chemical changes.
You can put proteins together to make an environment, a chemical environment, which act as machines, chemical machines.
The key factor I want to illustrate here is that all of these processes are producing lots and lots of different environments in which these chemistries can go on simultaneously and it's absolutely wonderful.
It's absolutely wonderful that that should work and that's the basis.
We see that structure at a higher level which does not reflect that complexity.
If we look at electron micrographs of cells, and this is a picture of a cell from a standard textbook which gives you some sense of that compartmentation, some sense of that spatial organization.
But that is not enough because if you have all these chemistries separated into different microenvironments, the whole system cannot work.
It cannot work because a cell isn't made up of a bag of a thousand or more different reactions.
It's made up of a bag of a thousand different reactions which interact and talk to each other and are regulated to produce purposeful behaviors which are the basis of all phenomena that you see in a cell.
How can that come about?
It could only come about if the microenvironments, which was identified here, can talk to each other, if there is signaling from one part to another and I've tried to illustrate that here very simply.
A chemical reaction that can only occur here must, in some way, be able to communicate the chemical re actions that occur elsewhere.
Otherwise, whole system cannot be coordinated as a whole.
So you have to have specialized ways of communicating from one environment to another so those chemistries can actually be coordinated and that can occur very locally as you see here at the regulation, or it can occur at a distance, or it can occur between cells.
All of these reflect organization which separates and connects by signaling and communication different areas of space within the cell but that's not the only way to separate chemical reaction.
You could also separate them in time in the light of the cell and that I've tried to illustrate here where a reaction that occurs here at TN could be different from a reaction that occurs at TN plus 1.
What I mean there is let's take a cell when it's reproducing itself.
The chromosomes, during DNA replication, carry out one set of chemical reactions which are quite different from chromosomes which separate during mitosis and it's the separation in time which allows those chemistries to actually function in different environments.
You don't have to separate in space simply.
You can also separate in time.
Now this is very important because the point I'm emphasizing here is that when you think of life as chemistry, it is a very special organized version of chemistry which is not found anywhere else except inside a living cell.
And what you need to do is to have a proper communication and proper regulation for that all to work.
So life is chemistry is, in fact, a sophisticated idea and it acts as an introduction to my last idea which isn't yet either fully formulated or necessarily fully accepted and this is the idea that there is something very interesting about biological organization and what it means and that's the last idea that I want to finish with.
So if you're having trouble and beginning to feel a little bit weary, just feel heartened we're with getting near the end, okay?
So just sort of, you know, have a big deep breath now and you'll feel better and we're nearly there.
Having said that, bits of these are a bit complicated, all right?
So you will have to concentrate.
[laughter] And some of it actually may not be right so you need to concentrate really well, just see where I'm getting it wrong.
Now this idea I'm very interested.
I want to consider-- and it really comes from, as I said, these thoughts about organ-- about the gene and also life as chemistry.
What is the nature of biological organization?
That's essentially the question I want to ask.
And I'm going to start just by sort of describing a couple of things that are important for biological organization and then I want to explain how I think we can get to grips with this.
And there's two characteristics that I want to emphasize.
The first is that biological systems that I've already emphasized have a sophisticated spatial and temporal organization.
They are organized in space, they are organized in time, organized in space in the ways that I've described.
Look at all of us around this room.
We all have characteristic shapes and those characteristic shapes, we see at all levels of biology from a cell up to ourselves.
In addition, biological objects are extended in time.
We persist in time.
We remain homeostatic in time.
We have temporal organization.
Again, you see that in its simplest form, the life of a cell.
A cell reproduces itself during the cell cycle.
It is temporarily organized during those changes that occur in the cell cycle.
So that's one characteristic of biological organization, organization in space and time.
The second crucial one is a concept that I think it was very well stated by Jacques Monod in a book called "Chance and Necessity." He defined what something he called teleonomic and by teleonomic, he meant purposeful behaviors.
That is, behaviors which appear to have a purpose.
Living systems are full of purposeful behaviors.
Purposeful behaviors, for example, are homeostasis, when something regulates to remain the same.
If you communicate either within a cell or between a cell or between different tissues or between organisms, communication is purposeful behavior.
Adaption and adaptation that you see in evolution appears purposeful.
Reproduction is purposeful.
So, living systems appear to have a purpose.
In fact, Monod had this nice example of that.
In the sense, he said, "Okay, let's imagine that Martians invaded the earth and they met living things.
How would they define living things?" So I told you how Monod would do it.
He would have this historical interpretation.
And what Monod suggested is that the chief Martian should ask the Martian troops is, "Do you see anything that has purposeful behaviors, behaviors with purpose?' Now occasionally, you can see in the inorganic world examples of where there may be purposeful behaviors and this interesting ideas around the [inaudible] for example which some might want to discuss perhaps later.
But essentially, this is something that you see in living things.
So when thinking about biological organization, I think we have to have central in our mind how we organize things spatially and temporally, how do we generate purposeful behaviors.
Well, I think the key issue with explaining biological organization is to consider living things, and I'm going to focus on the cell as the simplest unit of a living thing, as essentially not just a chemical machine as Loeb argued but as a logical and informational machine, an object which is processing information which is logically based and I'm going to suggest that our understanding of life which will be much improved if we consider living things in terms of the processing of information and the logic of how they are put together.
And I'm going to give you an example 'cause this is not a new idea.
It's one that, in fact, was very core to the beginnings of molecular biology, for example.
Think about the DNA molecule.
Now all of us think it's a beautiful molecule, the double helix, right?
Now it looks pretty, I agree with you, but that is not the reason why a DNA molecule is beautiful.
The reason why a DNA molecule is beautiful is what it tells us about information, how it's encoded and how it's passed on.
DNA is made up of a string of letters, an alphabet that spells sentences.
Those sentences encode information through DNA and RNA protein as I've explained through the central dogma.
In other words, to understand the significance of DNA for living things, you have to consider it in terms of it acting as a logical machine, encoding and expressing information.
Then it comes alive in terms of understanding biology and in this case particularly heredity.
Let me give you another example which is also obvious, gene regulation.
Jacob and Monod invented the idea of negative feedback in gene regulation.
What they described, it's true from the abstract genetic experiments from biochemistry, but what they described is you have a gene, you have a regulator that controls that gene expression.
The gene makes a protein and eventually that protein somehow associates with the upstream region and regulates the expression of the gene.
Now that, when you describe it as chemistry, is not interesting.
It's proteins binding to bits of DNA.
It only becomes interesting when you consider it in terms of the information that is generated by those chemical interactions and structures.
It generates a negative feedback loop.
That is, if the gene is overactive, it will produce something that switches that gene off.
It's an example of James Watt's governor on a steam engine.
You know, this thing with two brass cores that spins around and spins out and controls the amount of steam that goes into an engine.
That is a negative feedback control system just like we see in cells and it's a way that processing information is a logical module.
And what I'd really want to argue is that we should apply a similar approach to all activities of life and we should consider life and cells in particular as logical and computational machines and a real objective that we have should be to understand processes that are going on in these terms.
Let me give you just a couple of examples which are straightforward.
Regulation within a cell's signaling networks can be reduced to what we've seen here as circuits.
If you take an electronic engineer, they, in describing a radio which is what we have here, will have symbols describing different elements and components of the radio and that connection of those components would generate circuits that produce purposeful behavior, in this case the-- a transformation of radio waves into sound that you can listen.
Electronic engineers have principles so that they could look at a circuit without understanding all the details of the exact quantities a transistor has or a resistor has and they could say this will work as a radio and I'm going to suggest that that's exactly the approach we should use in understanding networks which are the basis, I think, of most things that go on in living cells exactly in these terms.
We have to workout how to connect them together to product this source of behaviors and it's going to be very rich behavior in cells.
I'm just going to give you a complete example, just two since the time is going late.
First of all, when we think of sig-- signaling, usually people think of something about this.
[Inaudible] A to B to C to D to E to F to G. This is how men think actually, okay?
[laughter] We'll come to that in a moment but you switch something on and then something comes out at the end.
This is actually how women think but we'll come to that in a moment.
[laughter] Just thinking of it as a static process like this throws away the dynamics of signaling in living things.
Now, Samuel Morse invented the Morse Code, okay?
And he took a telegraph where which originally as you press a button, then a light lights up on the other side of the room and that's a yes/no signal and he realized if he puts dynamics into that, you know, simply having a long burst of light or short burst, you can then write the works of Shakespeare, okay?
So, introducing dynamics moves you from a yes/no to the works of Shakespeare, just introducing that one dynamic.
This is immensely important.
I mean you see, the rich instead emerges and that I'm trying to put up here.
Signaling that has dynamics in it is hugely rich and we have yet to get to the grips of this in living things but this is crucial for understanding, I think, the logic and of the circuits that are operating in living things.
The second one I want to point out here is that this is how we think circuits work in cells.
This is how I think they really do operate, okay?
This is the male/female thing.
Now this is very, very interesting to me because this is intuitive.
You know that if you switch this on, this will be switched on.
I did-- I actually claimed-- if you-- if I ask what happens if you switch this on, what will happen out here you will not know, okay?
You will not know.
It is not intuitive if you cannot work it out and because these systems arose by evolution by natural selection, there are immense redundancies and add-ons which make it difficult to sort out what's happening and this is going to be one of the complexities of sorting all this stuff out because it has not been designed in this way.
It's been designed in this way as an add-on to pre-existing systems.
So it will not be automatically intuitive.
Now what about these other issues that I've talked about?
Spatial organization, temporal organization.
Well, spatial organization, let's just say a few things about that.
What's crucial here are issues of scale.
If we look at for example certain small structures in cells like for example ribosomes, what we see are perfect little shapes, okay?
Ribosomes are these small.
Other machines have perfect shapes.
But they are generated by molecules stuck together like sort of [inaudible], you know?
And you-- there is no variation in scale.
Living things are not like that.
If you take a cell which is 10 micrometers across, it will organize itself.
If you make it 30 micrometers across, it will also organize itself.
It is scale invariant.
This is what the classical embryologists [inaudible] used to call regulation.
You could regulate the embryo so it would fit half the size, three times the size.
That is much more difficult to do.
You have to general spatial order over a size range which is beyond direct molecular interaction and that requires the same information or logical networks that we saw at the underline also signal transduction.
Now what I've got up here is a picture of, in fact, a very famous reaction, the Zhabotinsky reaction.
That's chemical reaction that generates order, in this case over a scale of milliliters which is derived from molecular interactions and it's based on the fact that molecules are diffused in different rates, that there's positive feedback inputs and that generates this higher level order.
We, if we're going to understand spatial order, are going to have to think about the logical processes, the organizational processes that can generate this sort of spatial order and of molecular reactions.
In fact, this Zhabotinsky reaction which was anticipated by the famous mathematician Alan Turing who also formed the basis of computing, I have to say, in the 1950's, the-- in the-- this is a Turing equation which you can actually describe this.
It actually is probably not going to be very useful for biological form because, in fact, the scale of the-- of this order is invariant.
In other words, once you put in the constants in the equations, you will generate two or three millimeter phase length and you can't regulate it.
So, something more complicated is going on but I use it as an illustration, not an explanation but an illustration about we have to start thinking about these sorts of information.
Well, I'm trying-- I wanted to try and give you an example here of the sorts of problems we need think about if we're going to understand biological organization.
I think we have to emphasize the cell, we have to emphasize throughout as this logical and computational machine but I want to end in a way that my-- it sounds as if I'm sort of gradually going away on drugs actually [laughter] but I want to emphasize something which I think may happen and that is, that this complexity I believe will lead biology more and more from the common sense world that we are used to inhabiting into a much less intuitive world.
Now that has already happened in the physics.
There was a revolution that began in 1900.
Pre-1900, most of physics was understood in let's say the Newtonian paradigm.
It was a world that you could imagine, you could imagine billiard balls bouncing off each other.
You could imagine Newtonian laws of physics because it inhabited a world of three dimensions of space and time that we all saw as occupied.
At the turn of the 20th century, we had first Einstein and relativity.
Relativity is one of those interesting concepts where you sort of sometimes get it and sometimes don't, you know?
It's something where you're trying to imagine and you think you've just got it there, then you get a headache and it slips away, you know?
[laughter] And it's because relatively, it doesn't quite occupy our normal life.
You know, we don't see objects increasing in size.
We don't see time slowing down and speeding up.
It is not part of our normal experience.
We have travel dealing it.
But it's just out of reach.
Then you get to the 1920's, you will get to quantum mechanics and you are deep in Alice in Wonderland land, okay?
[laughter] And I really don't think anybody truly understands quantum mechanics in terms of visualization.
Occasionally at all, that people do.
I'm greatly heartened by my daughter who is a theoretical physicist and she tells me most of the time she doesn't understand it either.
But the truth is it's very, very difficult.
You've got to imagine two things appearing simultaneously in different parts of space.
It is not the world we inhabit, right, yet it is the basis of reality.
We have been taken away from our common sense world into a different world.
I mean Kant, actually Immanuel Kant argued we experience world in three dimensions of space and time and that's because that's the world we've been brought up in, that's the world we evolved in but it is not necessarily how reality is.
That's what he argued.
That was just simply philosophical and logic.
He said, "Space and time are subjective forms of human sensibility." It's just how we conceive of the world.
Now the physicists have had to abandon it.
I'm going to suggest that maybe the biologists have to abandon it too because understanding these complex networks and how they work is not part of our intuitive world.
It's not something that we can easily imagine and I wonder whether we will go into strange worlds where we need greater theoretical help as a biological profession from others who are more used to thinking about this, particularly the physicists and mathematicians because we are going to move from a common sense world, I think, into a strange world that we can no longer visualize.
I think it's very exciting but somewhat scary and on that note, I want to stop.
Thank you very much.
[Applause]
About the speaker
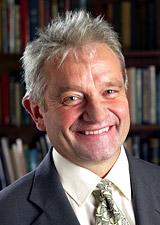
Paul Nurse
President and Professor, Rockefeller University
Nobel Prize in Medicine or Physiology, 2001
Sir Paul Nurse heads the Laboratory of Yeast Genetics and Cell Biology and is the president of the Rockefeller University. His research focuses on the molecular machinery that drives the cell cycle and cell division.
Born in 1949 in Norwich, Great Britain, he graduated from Birmingham University and in 1973 received the Ph.D. in cell biology/biochemistry from the University of East Anglia. After completing postdoctoral studies in Switzerland, Scotland, and Great Britain, Nurse joined the Imperial Cancer Research Fund (ICRF) in 1984 where he headed the cell cycle control laboratory. In 1988, he joined the University of Oxford to chair its department of microbiology. Five years later, he returned to ICRF as its Director of Research and later as Director General. In 2002, he was appointed Chief Executive of Cancer Research UK where he directed programs and support staff.
Nurse shared the 2001 Nobel Prize in Physiology or Medicine with Leland Hartwell and Tim Hunt for advancements in understanding of the cell cycle. This process is crucial to survival and mistakes can lead to tumors and cancers, and their research was hailed by the Nobel Foundation as revealing “new possibilities for cancer treatment.” Among Nurse’s extraordinary research accomplishments was the identification of a key regulator of the cell cycle in both yeast and human cells, CDK (cyclin dependent kinase). Scientists subsequently have identified half a dozen different CDK molecules in human cells and have found that higher than normal levels of CDK molecules characterize some human tumors such as breast cancer.
In addition to the Nobel Prize, Nurse has received the Albert Lasker Award for Basic Medical Research, General Motors Cancer Research Foundation Alfred P. Sloan Jr. Prize & Medal, Royal Society Wellcome and Royal Medals (U.K.), Pezcoller Award (Italy), Rosenstiel Award and Medal, Heineken Prize (Netherlands), Jimenez Diaz Medal (Spain), Jeantet Prize (Switzerland) and the Gairdner Foundation International Award (Canada).
A fellow of the Royal Society, Nurse is a member of the Council for Science and Technology, which advises the Prime Minister and the Cabinet of Great Britain, the European Molecular Biology Organization (EMBO), the Academia Europaea, the U.S. National Academy of Sciences (foreign associate), and is a founding member of the U.K. Academy of Medical Sciences. He was honored with knighthood in Great Britain in 1999 with the Legion d’Honneur (France) in 2002.
Nurse is an avid motorcyclist and glider and power pilot, with strong personal interests in astronomy, bird watching and natural history.