
From Mad Cows to Psi-Chotic Yeast: The Bizarre Biology of Prions
Transcript
Sydney Brenner won the Nobel Prize this past year.
David Baldwin, Bob Wineburg, [inaudible], Joe Stikes, and of course, tonight's speaker, Susan Lindquist.
These speakers in their work, that they have pioneered to epitomize [inaudible] past research interest and contributions that Ef and his laboratory have made over several year's time in medical research.
I know many of you are well familiar with Ef's remarkable life and the anecdotal stories of how his first love was art, which led to enter the Austrian Academy of Art.
Then responding to another calling, he would then go to medical school in Vienna and start what would be truly an outstanding biomedical research career.
However, throughout his life Ef's love for art would persist.
He was a prolific painter.
Many of you have probably seen his paintings in the biotechnology building and various buildings throughout town, and for that matter different research institutions and universities across the country.
Throughout his life, Ef would combine art with science and in a truly seamless fashion and certainly with an extraordinary insight.
And in both arenas, he was truly prolific.
Ef's scientific interests were directed at understanding the fundamental steps of a variety of biochemical pathways including oxidated phosporylation neurotransmitter release, mechanisms of enzyme catalysis, control of cell growth and really biochemistry of cancer.
After a distinguished career at New York University and then at the Public Health Institute in New York, Ef moved to Ithaca in 1966 as the Albert Einstein Professor of Biochemistry and as the chair of the then section of biochemistry.
He was an inspiration to a number of outstanding individuals, students and post docs who had trained with him and moved through his lab and go on to really establish their own laboratories.
And in all of this he was a wonderful mentor and a wonderful friend.
It's really even after ten years since his death, it's hard to contemplate how much he meant to this institution in terms of biology and he's still someone that is sorely missed.
Over the spirit of Ef's scientific breathe and accomplishment, we'll live through this lecture series.
And that's why we're delighted to have Susan Lindquist with us tonight as this year's Ef Racker lecture.
So David Shalloway will now introduce Dr. Lindquist.
Susan Lindquist received her BA from the University of Illinois and a PhD from Harvard in Biology, then served as a post-doctoral fellow at the University of Chicago where she rose from assistant professor to full professor of molecular genetics and cell biology and was appointed a Howard Hughes Investigator.
She joined MIT this year as professor of biology and is director of the Whitehead Institute of Biomedical Research.
Susan is widely known for her exceptional research on the biochemical mechanisms and biological consequences of protein conformational changes.
This research is of fundamental biology significance and, as we will hear tonight, is important for understanding human disease.
Her science is focused on three aspects of protein conformation and function.
When does a quality control system involved in proteins known as chaperones that are dedicated to helping other proteins fold to assume their proper functions?
Like human chaperones, protein chaperones prevent highly-interactive immature forms from making bad connections and arrest them apart when they do.
This is particularly important when certain types of misfolded proteins influence others to do the same.
These renegade proteins, prions, amyloids, are a second focus on Lindquist's research.
They have adapting variety of effects.
Some cause ghastly neurodegenerative diseases while others endow cells with new functions.
Their clear focus is signal transducing proteins.
Dr. Lindquist's honors are numerous.
Most notably she's been elected to the National Academy of Sciences, American Academy of Arts and Sciences and was appointed the Lasker Professor of Medical Sciences.
We're delighted to have her discuss tonight from mad cows to psychotic yeast, the bizarre biology in primates.
[ Applause ]
Thank you.
It's truly a pleasure to be here.
I have many friends and colleagues here who I've known over the years and it's a wonderful treat to see them again.
It's wonderful to meet some new folks and make some new friendships.
And I'm particularly honored by being in this illustrious company of this Racker lecture and honored truly by doing something for the memory of Ef Racker.
He's truly an amazing figure in molecular biology about chemistry and cell biology even.
His work has extraordinary breadth.
He is spoken of with true reverence for the quality of his work, the depth of his work, the variety and importance of his work.
Also what's lovely about this particular man is that he's also spoken about with great affection by everyone who'd known him.
So it's truly a pleasure.
[ Pause ] >> So there's some cows standing on the field.
And one says to the other, I'm getting kind of worried about this mad cow stuff.
Just heard that took old Bessie down to Johnson's farm.
Last night the OK Coral just lost half its herd.
Third one says, gee, fellas, I don't know what you're so worried about.
It don't effect us ducks.
[ Laughter ] >> So sorry about that.
I tell that terrible joke as actually to illustrate, my daughter got me that joke.
I tell that terrible joke to illustrate, I think, of something-- a very interesting phenomenon.
There are more mad cows jokes out there, if you can believe there are there are whole websites devoted to mad cow jokes and I get them from my friends all the time.
I particularly like that one just because my daughter got it for me.
But anyway, I think the reason there are so many mad cow jokes is because it's really a very frightening subject.
And I think it's a very natural thing for human beings to use humor when confronting something that is very strange and bizarre and that kind of scares them.
This is a photo page from one of my hometown newspapers.
I'm from Chicago.
If you can't tell from my accent, you probably will be the end of the talk.
My mom knows that I work on prions now and my mom is [inaudible] about [inaudible].
This is a disease that has a very bizarre ideology.
The mechanism that causes this disease is, we think we found a different one than most other diseases.
And it's really not very well understood.
But it is clear that it has left the species very [inaudible] from cows to humans.
This is a grim picture of the wholesale cremation of cattle that took place in Great Britain after the recent mad cow epidemic.
And as you all know, it had incredible economic problems and very big political repercussions.
But the thing that's really very frightening about this disease is that it's normally in humans, a very rare disease.
It happens spontaneous as I'll mention in a moment.
And you can get it in an infectious form.
You can get it from having mutations.
But it's really normally one in a million people get this disease.
It's a very rare disease.
And it's usually a disease of the older length.
The peak age for getting it is about 70 years old.
But in Great Britain over the last few years, about 150 people, young people, contracted this disease.
And it's a ghastly dreadful disease.
And the odd thing about it is, is that it has an incubation period anywhere from five years to 40 years.
And so there's really no way of knowing just how bad the transmission from cattle to the human population is going to be.
It may actually peak at a few hundred.
It may peak at a few hundred thousand.
So it's quite a serious subject in Britain right now.
This is one of the first manifestations.
Can people see if I stand right here?
Okay.
>> This is a manifestation of the disease in its first known human form as an infectious disease.
These are members of the Fore Tribe in New Guinea.
And they contracted the disease from cannibalism, ritualistic cannibalism.
When Carlton [inaudible] was studying these people and realized this was in fact a transmissible disease, about 50% of the population of this tribe was dying from this disease.
And it was thought initially that it was due to some sort of a environmental exposure or some sort of genetic defect.
But he established that it was in fact infectious.
And the reason why it was difficult to establish that is that the incubation period was so long during that time when people ate brain and the time when they got the disease.
He established it by transmitting the brain, by injecting it from human brain into apes and established that apes could get the disease as well.
And then he also showed that it was really very tightly correlated, in terms of pathology, with a disease that had been know in sheep for many hundreds of years, which had no known and still has no known transmission from sheep to humans.
So why this is transmitted from cows to humans is not now clear, except that it does seem to be much more virulent form of the disease.
So the reason-- in order to tell you why this is such a strange disease, what I'd like to do is just take you back to remind you what the normal cause of most human diseases are.
And that truly has to start with talking about DNA.
DNA, as you know, is the genetic information of the code for proteins.
And proteins really do just about everything in our bodies.
They are the things that allow us to perceive light.
They're the signals that are transducer to our brain.
It's transistor proteins that tell us that maybe we would like to pick that apple off the tree, the muscles that power our movement to get the apple, the energy that fuels the cells is all done by proteins, the digestion, the eating, the everything.
It's proteins are us so to speak.
And so what the human genome is doing is coding for proteins.
And basically there's a code of simple nucleotides here, this double helix, which I have straightened out for you here.
And what it does is this particular pattern of nucleotides there will dictate how amino acids will be assembled into proteins.
And proteins are nothing but long strings of amino acids.
There are about 20 different amino acids.
And each one has a different kind of character to it.
What gives proteins their uniqueness is that some of these amino acids are highly-charged and like to be on the outside or interact with each other, others are greasy and like to be sticky and on the inside, some are bulky and some are small.
They all have different properties.
And we have twenty different identities to put in these places with a long string of amino acids, when that protein folds up into a structure, it has some really marvelously unique properties.
Each protein, in order to have that property, must fold at just the right conformational state.
And that problem of protein folding is going to turn out to be a very interesting one in terms of this disease and in terms of manifestations of similar kinds of biology and very different sorts of systems.
So how do we get disease?
Well, there's two ways.
Generally, we get disease from viruses or bacteria that bring their own DNA inside of us and code for their own proteins that are going to do what they want to do.
So here is, for example, a virus shooting its DNA into a cell, this almost admissive cartoon, but it's sort of what a simple idea of what a virus would look.
This is a picture of a virus injecting into a cell.
The other way we get disease is also mediated by DNA.
And that happens when one of our own sequences of DNA mutates into a form that's not right and will make protein that doesn't do what it's supposed to do.
So this is one of the first examples of a known mutation causing the human disease.
And actually, it was discovered at MIT.
It's the sickle cell anemia mutation.
And this simple change of the code from this to this is responsible for making the hemoglobin protein into an abnormal shape.
And when that protein takes on an abnormal shape, the cells that encompass this protein take on the abnormal shape and they get stuck in capillaries and that's what's caused this disease.
One little change in the nucleotide sequence causing a change in one amino acid in the protein causing it to change its shape and be really very dreadful.
Now the strange thing about the prions is that they can manifest in several different ways.
You can get this same disease in an infectious form, as I mentioned by eating brain.
You can get it in a heritable form by having one of those mutations in a particular protein that will cause the disease or you can get it spontaneously.
>> Now the odd thing is that the spontaneous forms and the heritable forms that you get from having a mutation can give rise to infectious material some of the time, not all of the time.
But much of the time they actually give rise to infectious material.
So that led to the hypothesis that the infectious agent is a protein.
And in fact, when Stan Cushner and other workers were purifying the infectious material and the byway I have to say for this purification was just a nightmare actually injecting.
Can you imagine purifying material, for example, from a sheep brain and then injecting it and then waiting a year, year and a half, two years to see whether the sheep would get disease?
So what Stan Cushner did was he managed to figure out a way to transmit this disease to rodents.
Once it was transmitted to rodents it was possible to do it much faster.
I'd say three months.
And to purify fractions and figure out what was in this infectious material.
And as it was purified, it seemed to be mostly made up of protein.
They couldn't find any DNA in it.
Unlike viruses or bacteria that cause disease, there was no nucleic acids there, or at least none that could be discovered.
And then [inaudible] was x-raying activation of genomes, which is a way in which you can tell the size of what the genome is.
And you could x-ray the stuff, and x-ray that stuff, and x-ray that stuff until you had killed any known virus on the planet and it was completely unphased.
The stuff was just not destroyed by radiation.
So now is for the thought that this might be a protein, but how could a protein possibly come into a body and make more of itself and then transmit to another individual?
Well, part of the explanation that made this at least believable was that it's one of our own proteins.
So we're being infected by one of our own proteins when we get this disease.
And the way it's thought to be work is something like this.
There is a normal protein, prion protein, that's in our brains all the time.
In fact, it turns out to be one of the more abundant proteins in our brain.
It's a very abundant protein.
It's called PrP.
And it can get into this altered shape called the prion form.
This altered shape is not just an apparent shape that doesn't work.
It has an own property of being able to entice somehow or other proteins of that same type to change their shape too, and that's what produces more and more of the infectious material.
The protein's in our brains all the time.
It's producing more infectious material simply by converting stuff from a normal innocuous form doing whatever it's supposed to be doing, into one that can actually cause more and more proteins to change its shape.
And when it changes its shape, somehow or other it kills our brains.
Now the reason why I still have this down here is hypothesis, is because it's still really is hypothesis.
There's still many people who are skeptical about this, although I would say that the evidence is pretty darn good.
There's good genetic evidence, cell biological evidence and biochemical evidence from many, many different types of experiment.
But there are still some, these are some of the people by the way that made some really amazing contributions to this field, there's still some truly fundamental basic questions.
This is about the tenth effort in this field for many years that we don't understand.
For example, we don't understand what the function of the normal protein is in our brain.
So that makes it hard to kind of put together a whole story where you don't understand the function and you don't understand the change and the fact that it caused diseases because you're losing the function that's normal protein and you're gaining a new function or what is happening?
What is the function of this protein?
We don't know.
We don't know what the nature of the toxic species.
Why would when it changes its shape does it cause disease?
And this is the protein that's changed and altered and is capable of self-perpetuating that causes disease or is it some other intermediate?
And we have no clue as to how this ever gets started spontaneously in the first place.
How did it ever arise?
So I think until some of these questions are answered it's going to be harder for people to completely accept this hypothesis even though a growing number of scientists who have really studied the literature on this, do believe it and certainly the Nobel Prize Committee believed it because they awarded the Nobel Prize to Sam Printer [phonetic], as you all know a couple of years ago.
I entered this field because I was working in the yeast Saccharomyces cerevisiae.
And this is scanning electromagnetic [inaudible] of that organism.
It's a budding yeast and you can see that this is a mother cell and that's her daughter, it's a bigger daughter.
And I can say that I love my dog, but this is mad cow's best friend.
And the reason I can so that is that this organism is responsible for the three essential elements of civilization.
>> It is identified by the Romans, beer, bread and circuses.
And when I say circuses, I mean like your biology circuses.
Because we know more about this organism, it can do more tricks and more things to manipulate this organism, than we can with any other organism on the planet.
And so it's been the subject of an intense research effort and maybe a few members of the general public here may well know several uses of general [inaudible] biologists.
That's because people who are studying things like cancer, for example, growth control, how a cell decides if it's going to divide or not going to divide, those are basic mechanisms for making these very fundamental biological decisions are really very similar in this organism as they are in us.
So when we can study problems, these basic problems in this organism, it is a pleasure to do so because you can do so much with it so quickly.
And then you can translate that into higher organisms as well.
So I've been working on several organisms.
But I've been doing a lot of work on yeast for a long time.
And it turns out that there is a prion-life phenomenon operating in this organism.
In fact, we now know that there are at least six or seven prions operating in this organism.
There may well be 12, or 15, or at least 30 of them.
And in fact, I think it's a very, very widespread phenomenon in biology.
So what do I mean about prion in this organism?
In this organism, this phenomena of protein changing its shape and then influencing more and more protein to change its shape too actually serves as a mechanism for genetic inheritance, for inheriting a trait from generation-to-generation with no change in DNA.
And the way it works is basically very much like this sort of cartoonic diagriam.
We have a protein that can exist in not just one conformation, it's normal functional conformation, but another profoundly altered conformational state that's very stable but rare.
Well, once that appears it can influence other protein in the same-type to enter into that same state.
The reason it became a genetic element is that the protein is passed from the mother cell to her daughter when she buds.
So when the daughter's cells start making her own proteins, they're going to change their shape too.
And of course with that change in shape, there's going to be a change in function.
And so the cells may have a different metabolism.
And it's going to inherit that different metabolism from generation after generation after generation because of the change in protein shape with no change in DNA.
Now, with yeast we're lucky because we know the functions of these prion proteins, most of them at least.
We understand how the change in shape would change metabolism.
It's very simple.
It makes perfect sense.
We can replicate all this stuff in vitro a test tube, but purified proteins, all these changes in the shape and the ability of one protein to change into another protein, they can all be done in vitro.
And one of the reasons why that might be true is that this is not just a byproduct of a bizarre disease.
In yeast this seems to be a useful mechanism.
At least what I can say is that when cells have a particular protein that enters into the prion state we know that under some circumstances, they grow better than normal cells.
Other circumstances they don't grow as well.
But it seems to be an actually useful mechanism for cells to have one genome and to be able to have different inheritable and very, very stable states.
And in fact, you start thinking about the fact that there are ten of these or so, and the fact that you can have more than one of them in a cell and start turning into having lots of different stable inheritable states.
The particular prion that we work on is called Psi and it was discovered by Brian Cox about 40 years ago.
And just as the prions in cattle, and in humans, and in sheep mystified biologists for many, many years and the mad cows for many years, I can tell you that this Psi factor mystified yeast geneticists for just as many years.
It was just kind of strange.
They really did not have normal genetics.
And I can remember 15 years ago going to lectures with Brian Cox to talk about this Psi factor and hearing people say, why in God's name is he still working on this thing?
This is so just weird.
Why would anybody want to work on this?
And I think it's really a beautiful example of how really unusual bizarre biology can lead to tremendous new insights as long as the experiments are done really well and the experiment's unbelievable.
And so it was really a great thing that Brian did this work and as many colleagues that worked on it as well because it really laid the foundation for everything that we did and form actually some new insights into neurodegenerative disease as well.
So as you can see, these cells are red.
These cells are white.
When you streak these white cells out on a plate and you go up and colony these cells and you take that colony and streak that out onto a new plate and grow up colonies, they stay white.
These still stay red.
There's no difference in the DNA in these two organisms and these two phenotypes.
And these can interconvert.
Interconvert barely, about one in a million actually, about everyone in a million cells will spontaneously change red to white.
But generally it's really very faithfully inherited.
One of the-- there was this whole realm of odd genetics that was associated with this factor.
And I'm not going to get into that.
I'll just tell you one simple one.
>> When you mate a red cell to a white cell and then get progeny from those cells, with a normal trait that was encoded by the chromosomes of the nuclear genome, what you would see is half of the progeny would be red and half of the progeny would be white.
Not with this thing.
What you see here is that all of the progenate are white and it happens again, and again, and again.
It keeps taking over, white takes over.
And we now can understand that because it's really due to the inheritance of an altered protein shape so that when you mix one cell with another cell it's sort of a sexually transmitted disease.
You know, that's the only way, I mean, this is a disease.
It's transmitted by sexual contact.
But in other ways it's not really a disease.
It doesn't print the cells.
It just changes their metabolism.
Anyway, when there's the mixing cytoplasm, this will spread from one cell to the other and it will of course spread to all the progeny.
Now we could have originally, it was originally thought well maybe this weird spreading phenomenon was due to just a virus that's spreading or a plasma that's spreading or maybe the mitocondrol genome is spreading.
But in yeast it's quite easy to look at those things and no one could see any nucleic acid that was associated with this trait.
It just didn't make any sense.
And a whole series of experiments pointed to the idea that it actually might be due to a change in protein shape rather like the prion phenomenon.
And this is the protein that's responsible.
And I'm not even going to tell you all the details on the metabolism on the function of this protein because that's not worth getting into if I want to get into some other stuff too, which I want to do.
I'll just tell you that this protein has-- this is the cartoon of what the protein looks like.
It has a few different domains in it.
And this domain here is the functional domain.
When this is working, it turns the cells red.
Attached to it is this very weird-looking stuff.
And if you're a protein biochemist and you were to look at the sequence of this amino acids, look how all this protein is constructed, that linear string of amino acids, it is a weird-looking sequence.
It just doesn't look like a normal protein.
The very rich in glutamine for those of you that know what that is, it's kind of that little figure in terms of a human disease is a little [inaudible].
And it's also got some anti-charged amino acids here.
And it turns out that when cells are missing this part of the protein, they can never be white again.
That means this part of the protein is somehow is able to inactivate this part of the protein.
And if you don't have this around, you're always going to be red.
The other weird thing that associated initially from a genetic standpoint of this protein with that odd phenomenon, changing, switching to white and having the odd [inaudible] inheritance was that if you had this whole protein in the cell and then you came in and you separately expressed just this domain, over expressed that a little bit, you could trigger the cells to go into the white state.
And then they stayed white.
So you could stop expressing the protein anymore and just for a short period of time expressing this domain in the presence of the whole protein, which switched you to a new inherited state.
And so Reid Whitner postulated that maybe that was due to the fact that this domain here can fold into some funny way or it could modify this protein in some funny way so that when it's attached, it can activate this.
And once it gets going, it's going to keep on going for generation after generation.
It only has to be triggered and that's all.
So I first got into this because I've been studying as David said, protein chaperones and protein remodeling factors, things that change protein conformations, and we discovered that there was a particular protein remodeling factor that has the capacity to actually take protein aggregates apart.
It's called heat-shock protein 104.
And the reason it's called heat-shock protein 104 is that it's induced when cells are exposed to high temperatures where proteins start to denature and start to aggregate.
>> And it rescues them.
Without this protein you die if you're yeast cells are exposed to high temperature.
But if you had this protein, it 10,000 fold increases your vital because you can rescue your proteins from these aggregated states.
And we found, in collaboration with Gary Turnoff [phonetic] that if we expressed our protein remodeling factor in cells that were white, they would switch to red and they would stay that way for generation after generation after generation.
In other words, and the idea was that once this protein remodeling activity had gotten rid of this prion shape [phonetic] and the cell wasn't there anymore, none of the proteins were just going to fold, right, and do their normal thing and function and make the cell red again.
So the fact that you could, just by a short period of time sort of erase this heritable state in a cell by changing protein confirmation was a really strong piece of genetic evidence that maybe this was in fact a heritable trait due to a changing in protein.
But I can tell you that geneticists love this argument and biochemists thought we were nuts.
So we really needed to have some direct physical evidence that this was the case.
So I'd like to show you a little bit of the experimental evidence that John Glover, Marie Patino and JiaJia Liu in my laboratory produced to try to put this on a solider footing in terms of actually cell and biochemistry and molecular biology.
And I just want to show you a few of a myriad of different experiments that we've done and many other laboratories that have been working on this as well.
I think it's really important if you're going to have a lecture on science to not just hear what the phenomenon is, but actually to see how the experimental process of deciding on how something works takes place in science.
So the first idea is that maybe, we were saying that maybe there was some kind of heritable change in the state of this protein.
And we knew that this heat-shock protein could just aggregate things that were aggregated.
So we simply look to see whether there was a difference in the state of that particular protein and red and white cells, the sub-35 [phonetic] protein I just showed you.
Is that protein changing its state in the red and white cell?
And all we did, and this was really simple, we broke open the cells.
We spun them in a centrifuge and that's what happened.
And the red cells, how that happened, the protein was soluble.
It was floating around.
It was doing its thing.
It was working just fine.
When the white cells, we did that same experiment, this is what happened.
The protein was found in aggregates.
And those aggregates, because of what we know about the biochemical function of this protein, it can't function unless it's free to float around the cell.
So we did that in many, many different conditions.
We looked at lots of different cells and that always happened.
There where the cells were white the protein was in an aggregated state.
When the cells were red, it was soluble floating around and functioning.
Now, we can take those yeast genetic experiments that I was telling you about and make predictions about how you can change that state, that heritable state, and it'll change the physical state of the protein to go along with it.
So what we did was to take-- we're going to start with red cells and express this domain in them for just a little while, a few hours.
And we got rid of that extra expression, picked up a white colony and asked what had changed?
Has this physical property protein changed in those cells?
And it should have.
In this case it was soluble.
In this case it was insoluble.
What happens if we start with a white cell?
And now we, just for a few hours, over express that protein modeling factor.
It turns out that when you pick up a red cell, a red colony after that, the protein has changed its state.
Moreover, we looked at hundreds of other proteins.
No other protein was changing state like this.
This was the only one that we could find that was changing conformation.
But what we're really like to do, so we're [inaudible] what this protein could enter in an altered state and once it does it somehow or other can get other protein of the same type of change into the same altered state, in the active state too.
So how do we actually really see that?
And the answer came from this creature.
This is a jellyfish.
And Marty Chelthy [phonetic] several years ago, really revolutionized many, many of our experiments in cell biology by taking a protein from this creature, which glows green when blue light strikes it, and taking that green flourescent protein, or GFP, taking that protein and putting it onto other proteins.
>> It turns out that this protein that glows green folds really tightly.
It's a really well-behaved protein.
And you can stick it onto, by genetic engineering, you can stick it onto another protein and it will happily go around following that protein wherever its going in the cell and allowing you to visualize in real time where a particular protein is going and what it's doing.
And so when we put that protein, that green florescent protein, on the end of our protein we in fact found that in red cells is was completely soluble.
But in white cells, as soon as we started to express this, as soon as we could visualize it, it had in fact been captured by preexisting aggregates of sub-35 and we could visualize that these and others [inaudible] that these in fact were the sites where the previous protein that makes cells red had already aggregated.
It was literally-- you could watch it capturing the other protein.
We then decided to start doing some biochemistry on it.
And at first I will tell you that this was nightmare.
It was so frustrating and as we were trying to purify these proteins in various parts and pieces of the protein, they kept gunking up our columns and precipitating out solution and messing everything up and it was just a mess.
It's a protein biochemist nightmare.
And we decided instead of just giving up, we would look at the cell under an electron microscope.
What it turned out to be was not just gunk.
It was very highly ordered fibers.
So this region right here, which is the region, as I mentioned to you which is responsible for all this bizarre ability for this protein to change state and to capture other proteins and to get them to change state too, forms these beautiful [inaudible] beautiful if that sort of tells you the aesthetic plausibility of a biochemist here, which was not the case for Racker.
Anyway, it forms [inaudible], these 10 nm filaments here.
And these are amaloids.
And what do we mean by that?
They have a shape.
And it was kind of going like this in terms of talking about the shape.
They have that sort of a shape, you know, a stretched out shape where the protein chain folds in a very tight fold back and forth.
And you don't see that kind of a fold very often in biology.
You see filaments a lot.
You don't see filaments that are made up of that kind of a fold very often.
One of the places where you see them is in several different neurodegenerative.
You see them in prion diseases and you see them in Alzheimer's disease.
You see them in Huntington disease and several other neurodegenerative diseases.
They're rare enough that in fact there's dye that will interpolate into these fibers because of the spacing on these protein chains.
There's a several dyes in fact that it will interpolate into those chains and it will glow brightly when you shine light on them.
And so pathologists have been using these dyes, dyes like Congo Red red for 100 years to stain the brains, to diagnose the disease that people died from, that died from neurodegenerative disease.
And this yeast protein is stating the same thing.
And it's highly selective because you stain the brain, you don't have that disease, you're not going to see this protein [inaudible].
The other thing that was interesting about this was the following.
This stuff formed is simple filament.
This stuff, filament is the same essential core with just a bunch of junk along the side.
So the idea would be that this might have a property of entering those highly, not just aggregating and precipitating on a solution, but entering into a highly-structured state that once it's in that state can capture more protein and this just comes along for the ride.
The reason why you lose the function of that protein is it's a coagulate solution and it can't do what it's supposed to do.
So this, just this simple picture helped to provide us a little piece of how the biochemistry of this transmissible genetic protein base element could work.
But to establish it more firmly what we wanted to do was see whether we could actually watch it change state in a test tube.
And so all we did was take this dye Congo Red and there's probably a lot of other ways and more sophisticated techniques too, but this is the easiest one to do.
And we took the protein in the soluble state and added it to a test tube and asked, when will we see this appearance of these filaments, these fibers, this amyloid material?
And it took a long time.
At first nothing happened.
And then after about 30 hours or so it started to build pretty fast.
And the really interesting thing was what would happen if we took a very small amount of pre-formed fibers and added them to fresh soluble material?
That's what happened.
So even in the purified, with the purified system this simple protein all by itself has this capacity to exist for quite a long time in the soluble state.
But once it enters in to this altered state, you can actually watch it capture the protein extremely efficiently.
So the idea would be that in normal yeast cells with all kinds of other proteins floating around, when a protein is soluble and working, it just stays soluble and other chaperones help it to stay soluble.
But once it has altered into this conformational state, it can physically [inaudible] property all by itself of capturing other proteins in that state.
So to establish that and to relate that biochemistry to the genetics we did lots of different experiments and I think the simplest one we did was the most convincing.
We went back to this state and then we went and we took those lysates, those yeast lysates and the total lysates of a red cell and the total lysates of a white cell and we added them to this reaction.
And if you added the total cell proteins from a red cell, nothing happened.
If you added general cell lysates from a white cell, that's what happened.
So in fact, the white cells do have this protein in the soluble conformational state.
It does have the capacity to see this purifying reaction.
So then we wanted to relate the biochemistry to a human disease.
And is there any similarity between the folding of the prion protein and this yeast protein?
Can this genetic mechanism from an organism a billion years apart actually be related to what's happening in mad cows?
So one thing we decided to see was whether there were mutations.
Remember, I told you there were specific kinds of mutations that you can have which will cause you to get this disease spontaneously.
And one of the major classes in the mammalian prion protein was that there's some little repeat sequences in the protein and if those repeat sequences are expanded the protein, you spontaneously get the disease.
So we thought, well, there's some little repeat sequences in the yeast prion and other than that, these proteins have nothing in common.
You don't have to worry about drinking wine or beer or eating bad cheese tonight.
You won't get the prion disease from the yeast cells.
But we thought what if we expanded these repeats in the yeast protein.
And what happened was the following, white cells stayed white, red cells stayed red.
But if in these red cells we create that mutation, the repeat expansion, spontaneously they entered into this new conformational state.
It was heritable and infectious in the sense that it kept on going from one protein to another protein and another protein.
So we think if there's something fundamentally similar about the underlying biology of the way in which these proteins change their conformational state in yeast and mad cows and we can relate that to the biochemistry in yeast because when we look at what happens when we just put that pure protein in the test tube, it has exactly the physical property you might expect.
What happens is that if this is a time course of a normal protein, it would be taking a long time to make its change in shape.
This protein with the repeat expansion spontaneously does that all on its own much more rapidly.
So this spontaneously causes this new metabolism to appear in a heritable way.
It also spontaneously enters into this altered kind of conformation in the test tube.
So since then we've been doing a lot of stuff and we've been, for example, putting-- whoops.
We've been putting other mammalian disease proteins now into yeast because basically the mechanisms that govern protein folding and protein misfolding are about as ancient as life itself.
They're very, very highly conservative and we thought well, maybe we can learn some important lessons about the ways in which proteins misfold and the quality control systems, how do they keep their normal shape, what happens when they get in an altered shape that might actually help us [inaudible] human diseases.
One of the diseases we're working on is Huntington disease, which for the scientists in the audience will remember that that's caused glutamines.
When glutamines stretch, expand, stretch glutamines, amino acids in that protein expand beyond their normal size, if you have one of those expansions, you will get Huntington's disease.
And the longer the expansion, the earlier you get the disease.
And again, all of these diseases in the nervous system actually are really ghastly diseases and they've been very, very hard to study.
So this is what happens when we put those proteins into a yeast cell.
We can duplicate the change in conformational state that's normally seen in neurons when they're dying in yeast cells.
We can change the conformational state with the same protein, HSP104, that we used to change the yeast prion back into its normal state.
We're also working on [inaudible] neucleon, which is the protein that causes Parkinson's Disease.
And depending on whether or not we express it at one dose per cell, at one dose per cell it's in the membrane.
Two doses per cell it comes off the membrane and aggregates.
And that's when the cells start to die.
So these are just cells that are not expressing the protein.
These are cells that express the empty vector.
And these are cells that express the [inaudible] at two doses.
It kills these cells.
And this gives us a mechanism.
We're now searching for-- pharmaceutical agents are searching for genetic mutations that might reverse the toxicity of this protein and help us understand why the protein kills and what might change it back into its known state because it's much cheaper and easier to screen a hundred thousand drugs on yeast cells than it is to screen a hundred thousand drugs on say, a mouse model of Parkinson's Disease.
Now of course, you might also want to know, what about the prion?
Have you done anything to study the mammalian prion in yeast cells?
And yes we did.
We had some actually quite surprising results there.
And that is the two mammalian cells to test the hypothesis that we arrived in this.
And it seemed to confirm it in mammalian cell types.
And the basic idea was the yeast cells were telling us that maybe the change in state that was taking place in the mammalian prion protein was not taking place where people had thought it was taking place.
Normally this protein is made in that [inaudible] membrane department of the cell and ends up on the outside of the cell.
And it was thought that the misfolding took place out here.
Our yeast experiment suggested to us that this folding was taking place here.
And we know that there's a quality control system that when things go wrong in here, the proteins are recognized as this is trouble and cells shoot their proteins out into the cytoplasm for them to degrade [inaudible].
And so we thought that maybe when something might inhibit the protozoan, it would cause this protein to accumulate in the cytoplasm and that might cause disease.
Or you might also get disease by having mutations that increase in this folding here that would increase the rate of the protein going in the cytoplasm.
So I'm not going to take you through all of those [inaudible].
And those terms are just about to be published in science.
In fact, they're online in Science Now but they don't come out in the print version yet.
If anybody does want to go through the details can.
But I'm just going to show you one thing and that was to establish whether or not our hypothesis that we were doing, that we were coming to from yeast and then testing in the mammalian cells [inaudible] tissue culture was relevant to the disease in the whole organism.
What we did is simply directly express that [inaudible] protein in the cytoplasm by not having it go into the membrane in the first place.
Does the simple increase in the rate per [inaudible] made in the cytoplasm cause prion disease?
And this is [inaudible].
These are two siblings, one of which was born with the gene that was [inaudible] in cytoplasm and one which wasn't.
And I think you can see pretty easily which one was.
This mouse has a very typical prion disease.
The brain pathology is very similar.
It's a little bit unique to this particular construct but overall, and compared to the infectious form, because the infectious form normally starts in one place and then it spreads whereas our is happening all over the brain all at the same time.
But at least is established-- has allowed us to go now from seeing something in yeast, really from what the people who are studying mad cow disease in mammals taught us something about how a totally new form of genetics might be occurring in yeast, a form of genetics in which you inherit a trait by inheriting a protein with a different fold rather than by-- there's no change in the [inaudible] and then taking the yeast cells, and understanding some things about how things are misfolding in yeast and then going back into mammals and testing our hypothesis there we think we found out something interesting about how the mammalian disease might work that would have been hard to actually find out by starting with mammalian cells to begin with.
And so that gives us now some new therapeutic strategies to try on these diseases because they're really absolutely incredible diseases and there haven't been any good therapeutic strategies yet.
>> So in terms of talking about-- finishing up by just comparing mad cows to psychotic yeast, there's lots of differences.
They're functionally completely unrelated proteins.
They're in different compartments of the cell.
One was first manifested as infectious and the other is [inaudible] mechanism of genetic inheritance but they have a lot of similarities.
They both are sort of the change in the state that's changed, that's associated with the change in phenotype is associated in formation of amyloid fibers.
We don't know that the amyloid per se is the actual disease-causing [inaudible] changing thing, but it leads to that it's one of the protein folding pathways that [inaudible] amyloid that does it.
Although peptide peeks are important in both cases, the main thing is that you have a solid perpetuating change in a protein confirmation that alters phenotype.
The thing I want to leave you with is the thought, we're beginning to actually have some evidence on this, that this actually may be a common, that is a normal biology, that there was actually proteins that could undergo these changes in state and do so as part of normal biology.
We think of the [inaudible] yeast that are part of the normal biology of yeast cells.
We actually are now beginning to find a much wider array of these proteins in these altered conformational states that once they're established can self-perpetuate.
One of them is a transcription factor.
Another is a protein that might be involved in establishing memory.
And in our experiments this is still quite speculative.
These experiments are not finished yet but we're beginning to get the feeling that this is just the tip of the iceberg in terms of how these protein folding states can influence biology.
I'll stop there.
[ Applause ] >> Thank you.
Yeah?
About the speaker
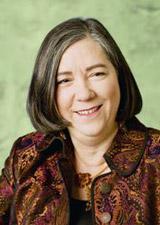
Susan L. Lindquist
Director, The Whitehead Institute for Biomedical Research, MIT
Susan Lee Lindquist received her Ph.D. in Biology from Harvard University and her B.A. from the University of Illinois, then served as a postdoctoral fellow at the University of Chicago where she rose from Assistant Professor to full Professor of Molecular Genetics and Cell Biology (1976 to 2001) and was also Howard Hughes Investigator (1988 to 2001). She joined the Massachusetts Institute of Technology this year as Professor of Biology and Director of the Whitehead Institute of Biomedical Research.
Dr. Lindquist is widely known for her exceptional research on the biochemical mechanism and biological consequences (including human disease) of protein conformational changes. Her numerous publications which frequently appear in Science, the Proceedings of the National Academy of Sciences, and Nature, are both rigorous and comprehensible to a broad audience. Her science has focused on three aspects of protein folding. One is the quality control system involving proteins known as “chaperones” that are dedicated to helping other proteins fold and assume their proper functions. Like human chaperones, protein chaperones prevent highly interactive, immature forms from making bad connections and wrest them apart when they do. This is particularly important when certain types of misfolded proteins influence others to do the same. These renegade proteins, “prions” and “amyloids,” are a second focus of Lindquist’s research. They have a dazzling variety of effects: some cause ghastly neurodegenerative human diseases, while others endow cells with new functions. The third focus is “shape changer” proteins, also called signal transducers. These maintain their general shapes all the time, but routinely switch a small region, folding and refolding, to drive growth and development. The chaperone Hsp90 stabilizes shape changer proteins that regulate growth. The genetic changes in these proteins are often too small to affect the organism on their own. But under stress, the interactions between Hsp90 and these proteins are disrupted, revealing hidden genetic changes that may not have appeared otherwise. Because some abnormalities might provide a survival advantage, the process might be an important force or “capacitor” in driving evolution.
Lindquist’s honors are numerous. It is of special relevance to note that she gave the Keith Porter Lecture at the 2001 American Society for Cell Biology annual meeting and was a keynote speaker at the 1998 ASCB meeting. She has been elected to the National Academy of Sciences (1997) and American Academy of Arts and Sciences (1998), and was awarded the Novartis Drew Award in Biomedical Research (2000).