Efraim Racker

Budding Yeast and the Brain
Transcript
[ Inaudible Discussion ]
- >> Richard Cerione
I'd like to welcome all of you to the- what's now the 12th Efraim Racker Lectureship in Biology and Medicine.
I particularly would like to welcome Ann and John, Ef's daughter and son-in-law and grandchild Caitlin.
They faithfully attend all of these.
I'd also like to welcome President Lehman and Provost Martin.
And really thank all of you for coming out of here on what's been rather interesting weather pattern today.
I-- I've assured to the speaker this is very atypical for Ithaca, nothing likely ever experiences in Berkeley.
This lectureship was initiated in 1992 with the intent of bringing both to the scientific community and to the general public a coverage of some of the major breakthroughs in developments in biology and biomedical research by those individuals who have been responsible for this major breakthroughs.
And in this regard we've been very, very fortunate in having some truly outstanding Racker lecturers including Jim Watson, Sydney Brenner, David Baltimore, Harold Varmus, Joan Steitz and of course this year's lecturer, Randy Schekman.
These speakers in the work that they have pioneered truly epitomized the breadth of Ef's research interest and the contributions that Ef and his laboratory made over the years to biomedical research.
Many of you probably heard the story that Ef's first love was art which led to his acceptance to the Austrian Academy of Art but he also had a deep calling in love for medicine and so he would also attend the University of Vienna Medical School.
And throughout his life, he would combine art and biomedical research interest in science.
In almost a seamless fashion certainly with an extraordinary insight and was quite prolific in both arenas.
Ef's scientific interests were directed in understanding the fundamental steps of a variety of biochemical pathways including oxidative phosphorylation, neurotransmitter release, the regulation of enzyme catalysis.
He pioneered the development of reconstitution approaches to put back together very complex biochemical pathways and he was very interested in the molecular mechanisms that underlie the control of cell growth in cancer.
After a distinguished career in New York University and at the Public Health Institute of New York, Ef moved to Ithaca in 1966 as the Albert Einstein Professor of biochemistry and the then chair of the section of biochemistry.
He recruited a number of outstanding biochemists and in that point Cornell was really a Mecca in bioenergetics.
He was also an inspiration to a number of outstanding investigators who were trained with him and go on to develop their own independent research careers and through all of this, he was a wonderful mentor and a wonderful friend.
It's now been a number of years since Ef died.
And the truth is we still have not come close to replacing his inspiration in this scientific presence in the life sciences here at Cornell.
But our hope is that Ef's spirit of scientific breath and accomplishment will continue through this seminar series.
And so we're particularly pleased to welcome this year's Ef Racker lecturer Randy Schekman from the University of California, Berkeley.
I should add that Dr. Schekman is also Cornell's first distinguished lecturer in cell and molecular biology.
This is a lecture series.
It's been sponsored by President Lehman and Provost Martin.
But the intent of this series being again to bring outstanding individuals in areas of cell and molecular biology to Cornell so that we can hear their works, so that we can share their thoughts in terms of where are likely to be future impacts and then trying to understand the fundamental workings of the cell.
And just maybe and maybe hopefully to gain a glimpse of the kind of scientific vision and passion that was so fully exemplified by Ef.
So again, it's a real pleasure to have Dr. Schekman here as this year's Racker lecturer and first distinguished lecturer in cell and molecular biology.
And he will be introduced by David Shalloway.
[ Pause ]
- >> David Shalloway
For almost 30 years, Dr. Schekman has been studying the cellular mechanisms that control the movement and secretion of proteins in yeast cells.
This may sound arcane until one recalls that secreted proteins include some of the most important signaling in control molecules such as insulin, growth factors and hormones, neurotransmitters, defects and their control are involved in a wide-range of diseases.
But why yeast and not something more interesting like us, humans.
As we teach in our programs, one of the most important skills of a biologist is define problems that are both important and also doable or more precisely define ways to pose important problems in doable context.
The jargon we often use is model system.
If it's too hard to study in one organism, maybe you can learn something from studying it in a related one that is simpler or where more powerful biological tools are available.
Yeast is a favorite because of the wonderful methods available for studying its genetics in biochemistry.
But it's easy to make mistakes.
You can study an exotic model system in great detail but in the end you find out that it doesn't pertain to the system of interest.
That's why Randy's interest in the '70s to study protein transport in yeast was courageous.
Obviously, it worked out.
And he went on to show that the mechanisms of protein secretion are evolutionary conserved in nucleated cells.
What you learn about yeast is usually applicable to the situation in human cells as well.
And yeast is important in its own right in biotechnology.
Beyond its well-known role in some popular beverages, it is one of the best systems for commercial production of vaccines, recombinant insulin and other biological compounds.
For example, the only commercially available hepatitis vaccine is produced in yeast.
And much of the scientific background needed to make this possible was provided by Schekman's work.
Dr. Schekman is currently a professor in the Department of Molecular and Cell Biology at Berkeley and an investigator in the Howard Hughes Medical Institute.
He's a member of the American Academy of Arts and Sciences and National Academy of Sciences where he is chair of the Biochemistry Section.
He has been the president of the American Society for Cell Biology and he is the scientific director for the Jane Coffin Childs Memorial Fund for Biomedical Research.
He has won numerous awards including the Eli Lilly Award in Microbiology and Immunology, the Rosenstiel Award in Basic Biomedical Science, and last year he received the Albert Lasker Award in Basic Medical Research, probably the most prestigious American award in medical research.
We're delighted to have him with us tonight to speak on budding yeast in the brain.
[ Applause ]
- >> Randy Schekman
Thank you very much David and Rick for your very generous introduction.
It's a special privilege to be here to honor Ef Racker.
In ways that the family probably couldn't appreciate, Ef Racker had a special role at a early stage in my career.
If I can take you back to before many of you were born, it was a Friday in October of the year of my PhD thesis defense, 1974.
I was a graduate student with Arthur Kornberg where I learned to do biochemistry and Arthur was very good friends with Ef.
Ef would come out occasionally to visit with Arthur and on this occasion, just by coincidence, he was at my thesis defense in the audience preparing for a lecture he was to deliver later that day and a symposium in which he was participating the following day.
Well, at the beginning of the thesis defense, Ef introduced himself.
I was greatly honored to have him present during this presentation.
And what I talked about was very much in line with what Ef believed himself, the analysis of complex biochemical reactions by fractionating enzymes.
So he appreciated what I was doing.
And I was honored when just before the end of my seminar, he stood up because he had to prepare his lecture and in front of everybody congratulated me for what I had done.
Unfortunately, I have to say that Kornberg for some reason wasn't quite as pleased with my presentation that day.
[laughter] In fact he was rather upset.
And so at the end of the presentation, we adjourned to Arthur's office with a thesis committee for what was at the time, some friendly conversation, a few polite questions, and a pat on the back and you're on your way.
Only on this occasion we got into a shouting match because of some perceived lack of appreciation on my part of what Kornberg contributed to my work.
[laughter] Very uncomfortable.
I was dismissed from the room thinking that my career was over.
And as I paced up and down the hallway awaiting my fate with a closed door.
Ef had just finished his preparations.
He saw I was distraught, he came up to me, he put his arm around me and he said, "Randy, I know how you feel.
Arthur yelled at me today, too." [laughter] So I deeply appreciated the role that Ef had in rescuing my psyche and possibly my career.
So, what I'd like to talk about today is an introduction to the work that we've been involved in my lab at Berkley for the last 28 years.
I'll do so in two lectures.
This evening's lecture is designed to be an introduction to the subject of protein traffic.
And I have weaved a tail in the context that something that we can all appreciate, and that is the human brain.
Tomorrow, I'm going to discuss in rather more detail the nitty-gritty of this process and so that will be for the [inaudible] and those who are particularly interested in molecular mechanisms.
But this evening I hope to appeal mainly to a broader audience and particularly to the students who may be here to try to give you a flavor for how one goes about tackling a complex problem.
And then the end you'll see how it was Racker's dictum don't waste clean thinking on a dirty enzyme that motivated our effort.
Well, here is the subject of our inquiry this evening, the human brain.
The subject that philosophers and poets and scientists and even humorous for instance, Woody Allen, considers this his second favorite organ.
How do we tackle the process of thought, how to plumb the depths of the human brain?
As a biochemist and specifically as a reductionist, I feel that one way to approach it is to try to take the problem apart into its component pieces.
And for at least 50 years, we have appreciated how cells within the brain communicate with one another by the transmission of electrical impulses and their movement between cells in the form of a cap of-- transmission of neurotransmitter substances that bridge signals from one cell to another.
We understand this from largely a cell biological description and we can see at the anatomical level a feature characteristic of the junction between nerve cells, in this case between a nerve cell and muscle cell, a characteristic morphologic landmark that is the business end of communication between nerve cells.
So let's focus our attention on the interior of this nerve cell, cross-section seen on the electron microscope depicting a pack-- a group of vesicles, little packets of information, each carrying chemical substances called neurotransmitters responsible for transporting a signal from a nerve cell, in this case across a junction called the synapse to a muscle cell.
We understand a great deal about the chemistry of this process, we know that these packets, these vesicles represent a quanta of information.
If a nerve cell is stimulated, a very small number of these packets or vesicles merge with the surface of the nerve cell and discharge their interior content to the synapse producing a chemical response that is detected by receptors, antennae on the surface of the opposing cell at the postsynaptic membrane.
Now we know a great deal about this process from the application of very sophisticated morphologic techniques.
And here for instance is a series of micrograph taken by a morphologist who studied this process, John Heuser, a very talented microscopist, who developed techniques to visualize the intermediates in the fusion of packets or vesicles with the synaptic membranes.
So if you follow the arrow in this sequence of events, you see a particle, a vesicle that is poised, ready to go primed for discharge, on the moment it receives its signal, the membrane of the vesicle and the membrane surrounding the cell merge and the interior of the vesicle is discharged to the outside of the cell.
And you can see this in a sequence of events resulting in the release of neurotransmitter, its diffusion across the gap and its recognition by receptors on the opposing surface.
We can also visualize this by another technique, another morphologic technique where we inspect the outer surface of the cell just as it is primed for discharge of neurotransmitters.
So here is an aligned view of the membrane.
So we're looking into the nerve cell, the nerve cell, an active zone where you're able to see beyond the membrane surface.
Here you would see vesicles poised and ready to deliver and it's possible to simulate this process and to visualize within a microsecond the appearance of dimples on the membrane, the craters here that you see that represent nascent events where the membrane of the vesicle has just touched, merged with the plasma membrane surface and the content of the vesicle is about to appear from the plane of the slide in the synaptic cleft.
Well, we know at a certain level a great deal about this process.
And yet at another level, at the level that satisfies the biochemist, we know very little.
We don't know at this point the machinery responsible for the recognition and fusion of a vesicle with the cell surface.
We'd like to understand the molecules that mediate this process.
We'd like to understand how these molecules are sensitive to signals that trigger the fusion process.
We'd like to know how the bilayers, the membrane bilayers, the vesicle membrane in a cell surface membrane, actually physically merge.
And we'd like to know how the membrane can somehow be retrieved back into the nerve cell to regenerate a new vesicle so that this cycle may be repeated many times over.
How to study this process.
In the mid-1970's, two approaches where developed.
One in my lab using genetics and the other in a not-- a biochemical approach, developed in the laboratory of Jim Rothman, then a young faculty member at Stanford University.
These two approaches were designed to discover and to characterize the molecules that mediate this process.
Now unfortunately it's very difficult to study this process in the human brain.
And for this purpose, when I started my lab in the mid-1970's in Berkeley, we decided to use a surrogate, a simple eukaryotic organism, Baker's yeast, where one could apply the techniques of a classical geneticist and one could also apply the techniques of the biochemist to bring the tools of molecular biology to bear on the study of vesicle fusion.
But why would one even consider using yeast, a lowly eukaryotic organism to study this seemingly higher breed function?
Well, let me tell you in the next few slides that there is an aspect of yeast cell growth that very faithfully reflects the images that you've just seen.
Well, here is a cluster of cells, by no means the human brain, scanning EM image about cluster of yeast cells.
This is a single yeast cell, the mother portion of the cell, and a bud that emerges on the mother's surface and grows during much of the cell division cycle in preference to the mother cell until at the end of a cell division cycle, the donor bud is as large as the mother cell, at which point the cells separate and the process is repeated.
When cells are provided with a rich environment of nutrients, they can grow and divide every hour and a half, very faithfully ad nauseam.
So it's a very efficient process.
One that allows the propagation of yeast cells on a fermentation scale to produce very large populations of cells, kilogram quantities, good for biochemical approaches.
But let's have a look inside of the yeast cell to see the anatomic feature that guided our efforts.
This is now a thin section taken through the middle of the yeast cell.
It's very similar in a way to the thin section that we saw in the second slide of the nerve cell, though you'll see that the interior of the yeast cell is very much more crowded than the interior of a nerve cell.
All those densely staining material that you see represents particles called ribosomes that are actively engaged in protein synthesis.
There are however organelles.
This is a structure called the vacuole.
It's the yeast equivalent of a lysosome.
There are thin strands of tubules underneath the plasma membrane, the surface membrane.
But our attention was drawn very specifically to a cluster of small vesicles congregating under the bud portion of the yeast cell, the very side of the cell that enlarges during this 1 1/2 to 2-hour generation time.
So we thought by analogy to what I've just documented in the morphology of the nerve cell that these packets or vesicles may be the yeast equivalent of synaptic vesicles that would allow a yeast cell to enlarge by a fusion process that is where the vesicle merges with the cell surface to engage the cell in a step of enlargement.
And so one can also look at the yeast cell by looking at the outer surface of the bud.
And here we see a dimple forming on the surface of the yeast cell and if you look very carefully at the surface of this membrane, you'll see small craters representing events very much like in the images that I showed a few slides ago which we suspected represent events where our vesicle in the cytoplasm of the cell has just joined and is about to discharge its content outside of the cell allowing this bulge to enlarge by the accretion of more and more membrane material.
I've used a cartoon to summarize our views.
This is a wild-type yeast cell.
We imagine vesicles are produced somewhere in the cytoplasm of the cell directed into the bud portion.
The vesicle will contain a surface envelope, a membrane and will contain proteins on its interior and on fusion, the vesicle membrane would join a cell surface membrane and the content, the interior content of the vesicle would then be expelled to enlarge a shell around the wall of the cell representing the yeast cell wall.
Okay, well, morphologically then, this set the stage for an analysis of the process of production, transport, and fusion of vesicles with the cell surface.
But how do we study this process?
Well, given that this is yeast, it's possible to apply the techniques of classical genetics to look for mutations that interfere with the production, transport, or fusion of these secretory vesicles.
But we have a problem.
Imagine that vesicles of this sort are responsible for growth of the cell.
Assuming that's true, a gene required for this final terminal event in cell surface growth would be essential to permit the cell to grow since a mutation that kills the cell can't be studied if the cell was dead.
We look instead for mutations that are conditional, that is-- that exert their effect at a non-- in a non-permissive condition, a typical technique that a geneticist use-- uses this to look for mutations that produce a protein that is perfectly normal at room temperature but which unfolds at a temp-- a higher temperature, for instance, body temperature, so-called temperature-sensitive mutation.
So, one of my first graduate students and I embarked on a search for yeast mutants that grow at room temperature but die at human body temperature, 37 degrees Centigrade.
And we screened among the collection of such mutants for those that block the enlargement of the cell surface and which correspondingly accumulate within the cell a large pool of enzymes that would normally be discharged to the outside of the cell.
This would be the yeast equivalent of a mutant in a nerve cell that failed in its ability to discharge neurotransmitter.
Well, we were rewarded for our efforts with a very large collection of such mutations.
And let me take you through a few images of some of the mutants we found.
This was-- the first mutant that we found was a very dramatic event in the life of my-- the life history of my laboratory.
We isolated this mutant-- we knew that it-- enzyme that were normally discharged in the cell exterior or that's accumulated in the cell but it wasn't until Peter Novick, my graduate student, examined these cells by the technique of electron microscopy that we have a visual impression of the dramatic impact of this mutation.
Now recall from a couple of slides ago that a wild-type cell has a very small cluster of a few vesicles enriched in the bud portion of a cell, the part of the cell that's-- that [inaudible] to grow.
But in this mutant called Sec1, when the cells are warmed the body temperature, the cell fills up its complete content with vesicles that continue to be made but fail in the terminal event of fusion with the cell surface.
Very dramatic effect.
And when you find something like this as dramatic as it is, you go back and repeat it like a monkey or a lab animal stimulating himself by constantly pressing the liver.
We went back to the lab and repeated this selection procedure over and over again until we obtain a very large collection of mutations in different genes that block the secretion process.
After about a year's worth of work, Novick assembled a collection of about a dozen different genes that is, at least 12 different proteins, each of which in its own way is responsible for this last step in the fusion of a secretory granule with a bud plasma membrane.
It became possible through the efforts-- the pioneering efforts of Gerry Fink when he was a faculty member here at Cornell to clone yeast genes.
And so we embarked on a cloning effort to identify the nature of the proteins whose mutation was responsible for this secretion block.
And we found after a great deal of effort and the effort of a number of other investigators that the genes responsible for this terminal event in the secretion process are highly conserved.
They have their equivalence in the human genome, indeed as you'll see in a moment, the-- many of the various same set of genes responsible for the discharge of a neurotransmitter-containing vesicle have their moral equivalent in the lowly yeast cell.
So, here's a close up view of the vesicles.
You can see in some of the images that the vesicles that accumulate have a membrane.
And here is a cartoon that depicts in a very superficial form the genes that we know now are required for this last event in the fusion of a secretory membrane vesicle with the plasma membrane of the yeast cell.
The names are irrelevant for this discussion, just consider the shapes and colors, and note that at the synapse, we now know from a great deal of further biochemical work that the very same-- in many cases, the very same genes and proteins are responsible for the fusion of a synaptic vesicle with the presynaptic membrane.
Indeed in some instances, it's possible to take the mammalian equivalent of a protein, one of these proteins, and to use yeast as a surrogate host to express that protein, and in some instances the mammalian equivalent will replace the yeast function.
So if you have a mutation in a SEC gene that blocks secretion in a yeast cell, sometimes it's possible to harness the human equivalent of that protein to express that protein in the yeast cell and to have the mammalian protein functionally replace the yeast protein.
So this is an example, one of many of examples that we now know of that demonstrate the physical, functional, and evolutionary conservation of this process in all eukaryotic cells.
Well, that was the last event in the secretory process, but when we continue to inspect cells by this technique of electron microscopy, we found a number of other organelles whose transformation within a pathway was blocked at different stages in this process.
So, for example, another mutant that Novick isolated, blocks-- transport from a station preceding the formation of vesicles in this pathway, a structure that in mammalian cells is called the Golgi apparatus, a kind of a bus station through which traffic is diverted to different stations within the cell.
Now in a normal yeast cell, this compartment, the Golgi apparatus is barely visible, an occasional strand of membrane can be seen.
But when traffic through this station is blocked in this particular mutant, the structure continues to build up failing to form new secretory vesicles so that after about an hour at a high temperature and the cell that's about to die, the structure, the Golgi structure accumulates to a very large fraction of a cytoplasmic volume.
A very dramatic block out of about the midpoint in this pathway.
Yet, another phenotype was observed in a collection of mutations that block at the step preceding the one that I've just demonstrated.
In these mutants characterized by this example, we don't see vesicles accumulating.
We don't see Golgi stacks accumulating, instead we see tubules called endoplasmic reticulum, a good buzzword from AP Biology, for some of you undergraduates who maybe in the audience-- a structure that accumulates when traffic out of this organelle is blocked in a particular SEC mutant.
The structure winds around within the cytoplasm, it folds extensively under the cell surface membrane, and another sections, it is apparent that it makes direct continuous contact with the envelope that surrounds the nucleus, a structure that is found in all eukaryotic cells.
Oh, here again, a very large number of genes were identified, you see like an example of a higher magnification view, a very large number of additional genes were characterized, each required for some event in which proteins, membrane proteins, and small molecules are passed from this station to the next station in the pathway.
Here again, it was possible to clone the genes and in some cases to discover their function.
Now, I'd like to move into a little bit more specific territory to tell you what we've learned after we cloned some of these SEC genes.
One of the first genes that we cloned that had some important instructive value was a gene that was discovered by a brilliant graduate student in-- from my lab by the name of Ray Deshaies, a Cornell undergraduate on my lab, who developed a technique to identify the very first station in the process of secretion.
So, let's-- let me show you a cartoon to illustrate some of the stages that we've seen thus far.
We found a pathway that involves the transport of proteins through stations in the cell starting with a structure called the endoplasmic reticulum moving through small carrier vesicles to a structure called the Golgi apparatus in turn giving rise to new granules that are transported to the bud portion of the cell in which execute the final step in the pathway.
How does this pathway begin?
Where do the proteins come from that populate this pathway?
They originate largely on ribosomes bound to a site on the cytoplasmic surface of the endoplasmic reticulum.
And for many years, a number of investigators have explored the nature of this transit event.
How it is that a protein originating on a ribosome in the cytoplasm finds its way through some special portal in the ER membrane into the space, or into the membrane itself.
So, this is the entry way for the transport of all proteins that must pass eventually to the cell surface.
How does this occur?
A breakthrough occurred in my lab in 1987 when Deshaies identified a SEC gene called SEC61 which turns out to encode the major channel-forming sub-unit of the polypeptide assembly apparatus, an apparatus that is found in all cells even in bacterial cells.
Here is a depiction of the gene that Deshaies discovered.
If we examine the sequence of the gene we-- the gene is predicted to encode a protein that has 10 very hydrophobic membrane anchor domains, hydrophobic enough to allow this protein to weave in and out of the membrane.
It's a protein that itself stays in the structured ER, it does not move along the pathway because it becomes a permanent resident, a portal in the ER membrane, a channel through which all other traffic proceeds.
It folds in the membrane apparently to create such a channel and only this year, a fellow at Harvard Medical School, Tom Rapoport has succeeded in obtaining anatomic resolution crystal structure of a bacterial form of this apparatus, and one can see in this atomic resolution structure a channel of about 6 to 7 angstrom in diameter which almost certainly is the channel through which proteins pass as their being mated and enter the secretory pathway.
Well, in the yeast the process uses not only assembly as the protein is being made but it also uses the pathway that involves first the synthesis of a protein in the cytoplasm, and then its assembly in the membrane after the fact.
And one example that I'm going to tell you about in a little bit more detail now is a protein that it represents a mating pheromone in yeast.
So, yeast cells come in two flavors, A, an alpha, and alpha cells produce a pheromone that is secreted by the pathway that we've described that exits the cell and interacts with a surface receptor, an antenna on the opposing cell type allowing that cell to arrest and respond eventually to form a mating pair or zygote with the alpha cell.
This pheromone, this mating pheromone begins its life in the cytoplasm of the alpha cell as a soluble protein made by ribosomes which after the fact engages in a complex series of events and interaction with this channel protein, Deshaie's SEC61 protein.
It is conveyed by multiple contacts with other proteins eventually to pass through this channel which opens up to admit the protein allowing it to penetrate to the other side of the membrane.
Eventually it is managed, processed, and discharged in the cell surface.
Now, this protein, this mating pheromone is particularly convenient to study because it can be made in a biochemical reaction, in a protein synthesis reaction reconstituted in a test tube with isolated cytoplasmic proteins.
It can be made radioactive so that one can investigate as it moves from one station to the next in a test tube.
And in 1986, another terrific graduate student in my lab, David Baker developed a cell free system, a biochemical means of investigating this pathway, so that now we were no longer limited to the dissection of intact cells.
We could break cells open and recapitulate aspects of the secretory pathway allowing us the possibility of biochemical fractionation.
Here is an assay developed by David Baker and Michael Rexach, two students in the lab.
So what we want to do now is to investigate how this mating pheromone is handled by this pathway.
The first step in the pathway that I've just described is the protein progresses through a channel on the membrane.
It falls and begins its course within the cell.
The next step in the pathway is the protein is somehow recognized and encapsulated within a very small vesicle that buds pinches from the surface of this endoplasmic reticulum membrane.
We knew this was true from our electron microscopic studies, but if we were restricted to the electron microscope we wouldn't really understand the biochemical function of the SEC genes that are responsible for the formation of the vesicles.
So, the biochemist seeks to break this process down into its component stages eventually to isolate the relevant molecules and to put them back together in purer form to investigate how they work.
So for this purpose, we needed an assay and the assay is depicted on this slide.
What Baker and Rexach discovered was a means of breaking yeast cells very gently, so gently that the ER membrane remains in very large envelopes, so large that they will centrifuge to form a pellet with a very brief centrifugation.
So if you take this very gentle lysate of yeast cells, and you put it in a centrifuge, and you turn the centrifuge on and turn it off immediately, all of the ER being large quantitatively pellets.
The pheromone, the radioactive pheromone that is introduced through the Sec61 channel into this membrane similarly will pellet very rapidly with a brief centrifugation.
However, if these membranes are mixed with crude cytosolic proteins or as you'll see in a moment with a pure set of proteins, in the test tube, we see within minutes the formation of very small vesicles that pinch from the surface of the ER membrane.
These vesicles are so small that they cannot be centrifuged by normal centrifugal forces.
One must use a higher centrifugal spin to collect these membranes in a pellet fraction.
So, it becomes possible then to assay biochemically the formation of these vesicles with this simple incubation.
We look for the formation of small slowly sedimenting vesicles when proteins, soluble proteins are incubated with isolated large ER membranes and these can be readily separated in a centrifuge tube.
We simply siphon off the supernatant fraction and count the radio activity that remains within vesicles in the supernatant fraction.
So that is then an assay.
It's a biochemical assay that allows one to quantify and ultimately to purify the proteins from this crude cytoplasm that are responsible for the budding event.
We did this and over the course of several years, the students emerge from a cold room with three protein fractions in hand or I should say, in test tube, in a nice bucket, that when mixed together recapitulate this process.
No longer that we have to add this awful crude cytosol, instead we could reconstitute the process with a set of pure proteins.
Now, interestingly, when we mix these pure proteins with isolated ER membranes and we use a form of energy, GTP, a particular form that cannot experience hydrolysis, that cannot experience hydrolysis of a beta, gamma-phosphate bond.
We found that the proteins responsible for budding remained stuck on the surface of the vesicle.
This became particularly apparent.
Yes, when we inspect it, again, by this technique of electromicroscopy, the vesicle products that bud from the surface in the ER membrane, this is a low magnification view showing you a collection, very large collection of all the vesicles that bud, and if you look closely in this image, you'll see that every vesicle is surrounded by an electron-dense amorphous kind of fuzzy coat structure.
Buds-- have a closer look.
At higher magnification, this fuzzy coat structure becomes more apparent.
It says though, the proteins responsible for the budding event envelope a piece of membrane, grab this piece of membrane and pinch it off the ER and hold on in the absence of the ability of this form of GTP to be hydrolyzed.
Another view shows surface structure associated with the outer, the exterior of these vesicles.
Clearly some kind of structure is imposed on the membrane to deform it, to pinch it, and then to separate it from the ER membrane.
We can even show with isolated nuclei that the machinery responsible for budding pinches a piece of membrane directly from the nuclear envelope.
So, here is an example.
This is a thin slice through the yeast nucleus.
This is the interior of the nucleus with chromosomes that aren't readily apparent here.
This would be the cytoplasmic phase of the nuclear envelope and here are the two membranes that surround the nucleus.
The outer membrane of which is a substrate, a platform on which this coat proteins can assemble, pinch a membrane and separate it by fusion process from the donor ER.
But much of the work that I've shown in the last few slides had been done in collaboration with another scientist who happens also to be a great artist and his name is [inaudible].
I have a number of his watercolors.
One of which I've reproduced for you on this slide.
Here is [inaudible] in his garden in his home in Lake Geneva.
He claims in this image to be harvesting lettuce, but it looks very much like CAP2, they're like vesicles to me.
[laughter] And so, this will serve then as an entree to the-- a subject that I'd like to discuss in a little bit more detail and that is the nature of this code, a code that we call, CAP2.
So, we've spent some time investigating the proteins that comprise this code, and I'm going to tell you in the form of a snap shot in a little movie how we think they assemble on the surface of the membrane and how their assembly is coordinated with the recognition and capture of proteins that must be moved out of the ER membrane and moved to the next station, the Golgi apparatus.
And for those of you whore are particularly interested in the details, I will have a great deal more to say about this process tomorrow afternoon.
Well, here are the players, the molecules, the SEC genes whose biochemical involvement in this pathway we now know with some great precision.
The process begins with a small protein that binds the nucleotide GTP.
This protein is called SAR1.
The process extends by the application of another protein that has two subunits, a protein that is called SEC23 and SEC24 and these names [inaudible] from the genes that were originally defined in the collection made by Peter Novick.
The process of budding is consummated by a scaffold complex consisting of two other proteins called SEC 13 and 31 and you'll see in a moment how these molecules assemble on the surface of a membrane.
A landmark that says, "I am the ER membrane" is this protein, another SEC protein, a constituent of the ER that never leaves, it stays there.
It's a landmark.
It's a protein, too, which SAR1 is attracted, a protein that allows SAR1 to acquire its cargo of this nucleotide GTP, and the red and green molecules represent substrates in the membrane.
Some of which, for instance, these green molecules are designed to be recognized and to be moved out of the ER, to be moved along the way eventually to the cell surface and other molecules depicted here in red are designed to be ignored and to be retained in the ER where they will function and for instance creating the portal through which proteins pass on their way into this pathway.
Okay, the stage is set.
Now, let's have a look at CAP2, The Movie.
The process begins with SAR1 acquiring this nucleotide, GTP, landing on the surface of the ER membrane where the heterodimer 23, 24 binds.
This complex is then diffused in the plane of the membrane and by collision, sample, encounter and sample different molecules, only some of which the green molecules are designed to be packaged.
The scaffold protein binds to the surface of the membrane, pinches the membrane to deform it into the contour of a 70 nanometer vesicle, and the protein that binds GTPs are one all the while is hydrolyzing GTP to GDP in phosphate which renders the coat and scaffold unstable, so that the coat is shed revealing a naked vesicle that exposes membrane proteins on their way to the next patient in the pathway.
So tomorrow, for those of you are interested, we're going to explore in some more detail how these processes put together eventually to have a look at this process in atomic resolution-- in an atomic level of resolution.
But in the remaining minutes I'd like to tell you a different story that we initiated in the lab which came about by a rude awakening that I had some years ago, because when I turned 50, I received the following mailer from the-- oops.
[ Pause ] Yes. I received the following invitation in the mail from the American Association of Retired Persons early membership in the AARP.
So I was quite alarmed.
I thought this was a sign that perhaps I shouldn't be spending all of my time studying a process.
So unrelated-- so seemingly unrelated to the human brain.
And so around-- a few years ago, I decided to embark on a new investigation exploiting some of the information that I told you about as it applies to a very specific and very interesting problem in human health, and that is how the secretory pathway, in particular some of the events that I've just described, may be linked to the mishandling of a membrane protein who's maturation is responsible for Alzheimer's disease.
Now that seems like a bold and a difficult statement to support, but let me try to do so in the form of a few model slides.
So what is Alzheimer's disease?
There is the late onset sporadic form of the disease and then there are series of familial forms of the disease, both of which are related by the accumulation in the patient-- in the brains of patients with the disease, of plaques that are created by the mishandling of a protein called APP or amyloid precursor protein.
Let me tell you a little bit about APP.
It's a protein that's found on the surface, on the exterior surface of all mammalian cells, but it seems to have a particularly important function in the brain, although it's actual normal function is not really fully understood.
However, when this protein is mishandled by the cell then the process leads inevitably to the degeneration of very large numbers of nerve cells.
In the normal course of events, this protein experiences two cleavage events by enzymes, membrane enzymes in the secretion pathway that are referred to as alpha-secretase and beta-secretase.
So as we look at the fate of this molecule, bear in mind that this curly structure represents one part of the amyloid precursor protein, the part that is exposed on the exterior surface of nerve cells, and this other part is a part that's buried facing the cytoplasm of a nerve cell.
So these enzymes cleave to generate a piece of protein that floats away from the cell to produce another piece of protein that floats to the anterior of the cell, and then a stump that remains embedded in the cell surface membrane.
Ordinarily, this is a normal completely benign process having to do with the maturation of this APP protein.
However, sporadically-- sporadically, or in certain patients with a rare genetic predisposition, a sinister enzyme called gamma secretase acts in between the alpha and beta enzymes to insert another break in the backbone of the APP molecule producing an additional small bit of peptide called the Abeta peptide.
Now, why should cells care about this?
Well, there is one serious and pathologic consequence of the action of the beta and gamma secretases and that is to produce this peptide which doesn't live well enough alone, because instead, over a period of what can be years or decades, the peptide builds up into a plaque called amyloid, and this plaque inevitably leads to the degeneration of tissues in which it is embedded and is apparently-- because amyloid is apparently not disposed of in nerve tissue.
So what we like to understand in Alzheimer's patients, what gives rise to the production of this abeta peptide?
What unleashes the activity of the gamma secretase?
How do we study this problem?
Well, about a dozen years ago, certainly it was then already recognized that there are certain families that have a genetic predisposition to Alzheimer's disease, and it became possible in various laboratories around the world to identify the LOSI responsible for this disease and to pinpoint the mutations in genes that cause people to develop symptoms of Alzheimer's disease in their 20s and 30s, much earlier than the normal late onsets sporadic form.
Very interestingly, one particularly important gene was the APP gene itself that certain mutations in the APP gene predisposed to its illegitimate action as a substrate by the enzyme gamma secretase.
There are other proteins seemingly cooperating with APP to render it susceptible to this cleavage event.
And one of these molecules is called presenilin-- there are actually two presenilin genes, and what was particularly interesting to me a few years ago when I started to investigate this is that presenilin is a membrane protein that largely resides in the ER membrane and it appears to make contact in the ER membrane with the amyloid precursor protein.
So we've begun to explore this possibility using the vesicle budding reaction that I've described.
Now, how can we do this?
Yeast cells don't get Alzheimer's disease.
If you attempt to express APP and presenilin and yeast, they are not handled in anyway like they are in the mammalian cell.
However, we can rely on the fact that the machinery responsible for vesicle budding is evolutionarily conserved.
Indeed it's possible to take the yeast proteins that are responsible for forming vesicles and to mix them with mammalian ER membranes and to recapitulate over a billion years of evolution a union-- an unholy union between the yeast proteins and the mammalian ER to faithfully replicate the vesicle budding process.
So, postdoc in my lab, Gino Kim [phonetic], has embarked on an investigation using CAP2 proteins, the code proteins responsible for vesicle budding to explore the coordination in packaging of presenilin and APP into vesicles that bud from ER membranes harvested from the [inaudible] derived cultured mammalian cell lines.
We have cell lines that have wild type APP.
We have other cell lines that have mutant, presenilin mutant, mutations, where the mutations are introduced to reflect the very mutations found in human patients with the disease, and we're hoping to identify an influence of these mutations on the fidelity of packaging of these proteins.
And one-- maybe with one final thought, model, we imagine that in the normal circumstance, APP may form a complex with this membrane protein presenilin that permits access in the secretory pathway to the normal enzymes, the beta secretase and the alpha secretase, but perhaps not ordinarily access to the gamma secretase.
However, suppose we introduce a mutation into APP or in other patients, a mutation in the presenilin.
Perhaps, the complex forms but fails to protect that portion of APP that becomes a substrate for gamma secretase, in which case the complex is made that can be accessed by gamma secretase to produce and secrete this illegitimate peptide.
We hope to explore this process using the biochemical approaches that I've described which were made possible by the analysis of the SEC genes, and perhaps when I return to Cornell I'll be able to tell you in a later date what we've learned, but in the mean time very-- thank you very much for your attention.
[ Applause ] >> We have time for some questions.
[ Pause ] >> Actually, Randy, I have a question.
To apply that-- so-- it didn't say it directly but it's a pretty strong implication that the vesicle [inaudible] yeast had been adapted in by neurons later on in evolution.
Has that same vesicle pathway have been adapted in other places and in higher eukaryotes?
>> Yes, so the question is how widespread is this pathway, and in which tissues is it invoked?
>> And particularly for very specialized functions that you wouldn't have guessed that.
>> Well, wherever cells have vesicles that must be mobilized to the cell surface in secretory process is these genes are invoked.
The mammalian or other metazone equivalents have been discovered and where one can apply genetics for instance, in a fruit fly or the worm, the mut-- corresponding mutations in SEC genes have been identified and that very often they display lethal very, very often early embryonic lethal phenotypes or very specific neurological defects.
So, as far as we can tell, the pathway is concerned and exploited wherever cells need to secret proteins.
Yes, Tom?
[ Inaudible Question ] >> Yeah. I'm not aware of an example in yeast.
A membrane protein that is clipped as extensively as part of its maturation pathway.
There are, of course, examples as you know this mating pheromone that I've described the alpha factors is clipped repeatedly but all those cleavage events occur in the interior space of the Golgi apparatus, not even in the ER.
So, it's for that reason that I've decided that it would probably best not to try to recapitulate this process in yeast rather to extend our studies to neuronally derive cell lines and to rely on the apparatus that's contained within our cell and to exploit the evolutionary conservation only in some parts using these CAP2 proteins.
However, very recently, the complex of gamma secretase has been identified and reconstituted by the transformation of the yeast cell to produce an active gamma secretase that appears to work in yeast cells and perhaps in such a cell.
There are four subunits of this enzyme.
Perhaps in such a cell, one could, in addition reconstitute by transformation of the APP gene and then investigate how it's handled and the yeast transform it, but that hasn't been done.
Yes?
[ Inaudible Question ] >> Yeah.
[ Inaudible Question ] >> The answer is no.
Let me repeat the question.
Very good question.
So we can bud vesicles from the ER with these pure proteins.
What if we give it at a different membrane?
Will they blood vesicles?
And the answer is no.
And the reason is that the protein that allows Sar1to acquire GTP is only in the ER membrane, it's not in the Golgi membrane.
>> Okay, so this-- >> So, you have to-- but you can fool the system if you allow Sar1 to acquire a GTP by some [inaudible] which you can do in vitro, then you can bud vesicles from anywhere.
[ Inaudible Question ] >> Connection to what?
[Inaudible Remark] Yeah, so the question is we have this biochemical assay, how do we know that it's biologically relevant?
[Inaudible Remark] Yeah.
Well, the reason that we know that is that the genes were identified first by a genetic approach using leaving yeast cells.
So we had-- in order to discover this pathway, we first started with the cell and we asked the cell by using genetics, what are the genes that are responsible for this process?
The cell told us instead of genes that when mutant interfere with the process.
So, I think we can make the reasonable assumption then that from the genetics as a guide to the biochemistry that we must be following the correct pathway.
Indeed we can show when we take these mutants that are defective in vivo, we break the cell open gently.
The mutants that block vesicle budding in vivo also block vesicle budding in vitro.
In fact, that's how we can use that as an assay, biochemical assay to purify the wild type counter parts of the mutant proteins.
So, we have that link, I think fairly firmly in hand.
Yes?
[ Inaudible Question ] Yes. [Inaudible Question] Yes.
Yes. I've over simplified things, yes.
[Inaudible Question] Yes.
[ Inaudible Question ] Yeah, so the question is, do we know that the plaques that I have described are the real source of pathology in the disease, and the answer is that's just speculation on my part or on the part of lots of other people.
In fact, there is some evidence that the Abeta peptide long before it's formed this amyloid plaque may have pathologic consequences for the cell and there are other morphological correlates that have been described, some of which people feel more to have a more proximal role in the pathology of the disease you refer to fibrillar assemblies in the cell-- nerve cells of patients with the disease.
I have no idea from the literature which is more important than the disease, however, one knows that the proteins that I've described, presenelin, APP, are involved in the generation of this Abeta plaques and one can in fact recapitulate this process in mice by transformation.
One that's created mice that have the human mutations in presenelin and then APP and one can see in the brains of these mice plaques that developed.
Now, one doesn't know whether these mice are actually succumbed to Alzheimer's disease but there certainly is neurodegeneration going on.
So, I really admit that this may not be the whole story of Alzheimer's disease but certainly I think a very strong suggestion about a path to investigating molecular aspects of the process.
And so, lots of people as you know are investigating this and this is slightly different way of looking at it.
There's a question in the back?
[ Inaudible Question ] So that's a two-part question.
Is the ER polarized?
And there is evidence in-- certainly in mammalian cells for its regional specialization.
There are proteins that are uniquely in the nuclear envelope and not found in ER tubules that emanate from the nuclear envelope and conversely there are proteins in the tubules and they are not in the nuclear envelope.
Even within the ER tubules that are sub domains, there are some that have ribosomes found, there are others that don't.
So, to that extent there certainly is differentiation of the membrane.
Then you refer in your-- the second part of your question to a Brownian ratchet and I wonder if you're referring to a view on the mechanism of how a protein entry through the channel into the ER membrane.
Is that what you're referring to?
>> Yeah.
>> Yeah. So, there is a very interesting model that was proposed initially by my colleague, George Oster, at Berkeley, called the Brownian ratchet that he's applied in a number of different situations.
One of which is to the action of the channel itself.
So, in the channel, according to his view, the channel, the secretory channel, Sec61 is a passive portal through which proteins are transmitted vectorially and the orientation of the translocation event he suggest is guided by using a protein on the other side of the membrane that binds to the nascent chain and hydrolyzes ATP in a cycle of events, thus, biasing the diffusion of the chain to the interior of the lumen.
There is very good evidence for that in yeast and in mammalian cells.
So it's a viable explanation of the vectorial nature of the translocation machine.
>> One last question.
[ Inaudible Question ] >> Yeah. Actually, I don't know.
I'm not aware of any normal function of the Abeta.
And there certainly is a phenotype, a neurologic phenotype associated with null mutations of the APP gene.
They are not-- they don't produce an early embryonic lethal phenotype unlike the effect of a null mutation in the presenilin gene.
So presenilin seems to be more important for early development because it's probably involved in other pretty processing events.
APP deletion produces a viable but neurologically deficient mouse.
So, what it actually is doing-- what it's responsible for, which pathway is responsible for, I don't think is-- [ Inaudible Remark ] I'm not aware, I'm not aware of any normal positive function of Abeta but there may be.
>> Thank you.
David Shalloway: There'll be time for more questions in private at the reception just outside the atrium.
Let's thank Dr. Schekman one more time.
[ Applause ]
About the speaker
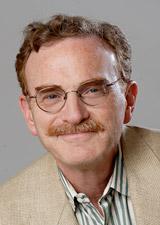
Randy Schekman
Professor of Cell & Developmental Biology, University of California, Berkeley
Dr. Randy Schekman is a Professor in the Department of Molecular and Cell Biology, University of California, Berkeley, and an Investigator of the Howard Hughes Medical Institute. He studies the enzymology of DNA replication as a graduate student with Arthur Kornberg at Stanford University. His current interest in cellular membranes developed during a postdoctoral period with S.J. Singer at the University of California, San Diego. At Berkeley, he developed a genetic and biochemical approach to the study of eukaryotic membrane traffic. Among his awards are the Eli Lilly Award in microbiology and immunology, the Lewis S. Rosenstiel Award in basic biomedical science, the Gairdner International Award, the Amgen Award of the Protein Society, the Albert Lasker Award in Basic Medical Research and the Louisa Gross Horwitz Prize of Columbia University. He is a member of the National Academy of Sciences and the American Academy of Arts and Sciences. In 1999, he was the President of the American Society for Cell Biology and was appointed Editor of the Annual Review of Cell and Developmental Biology. In 2002, he was elected Chair of the Biochemistry Section of the National Academy of Sciences and was selected as Scientific Director of the Jane Coffin Childs Memorial Fund for Biomedical Research.
Protein secretion is a fundamental and evolutionary conserved process in nucleated cells. Membrane traffic processes are responsible for cell growth compartmentation of metabolic pathways, and specialized activities such as hormone, growth factor, neurotransmitter, and antibody production.
Beginning in 1976, Schekman and his co-workers used genetic approaches to study membrane traffic in yeast cells. Ingenious methods were devised for the detection and enrichment of conditional mutants defective in the secretory pathways (sec mutants). Many genes were defined that chart a pathway of membrane transport and protein sorting that is remarkably similar to the pathway defined by cytologic means in mammalian cells. Schekman was the first to recognize that the mechanism of this complex but ubiquitous process could be dissected using a combination of molecular biology and biochemistry, and that yeast would be the simplest model system where such techniques are readily available.
Given the similarity in the transport processes across the evolutionary spectrum, the practical advantages of yeast fermentation have made this an important vehicle for the production and secretion of proteins important to the pharmaceutical industry. Recombinant hepatitis vaccine, the only commercial hepatitis vaccine on the market, is produced in yeast. One quarter of the current world supply of recombinant human insulin is produced by secretion in yeast. Much of the background for the applications was provided by Schekman’s elucidation of the secretory pathway in yeast.